Exploring Modelling Materials: A Comprehensive Analysis
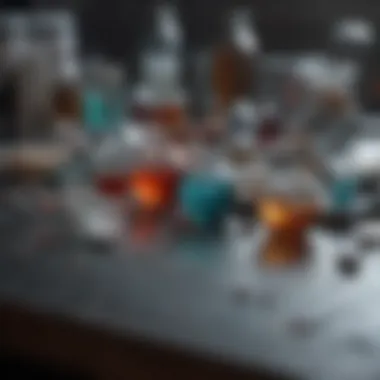
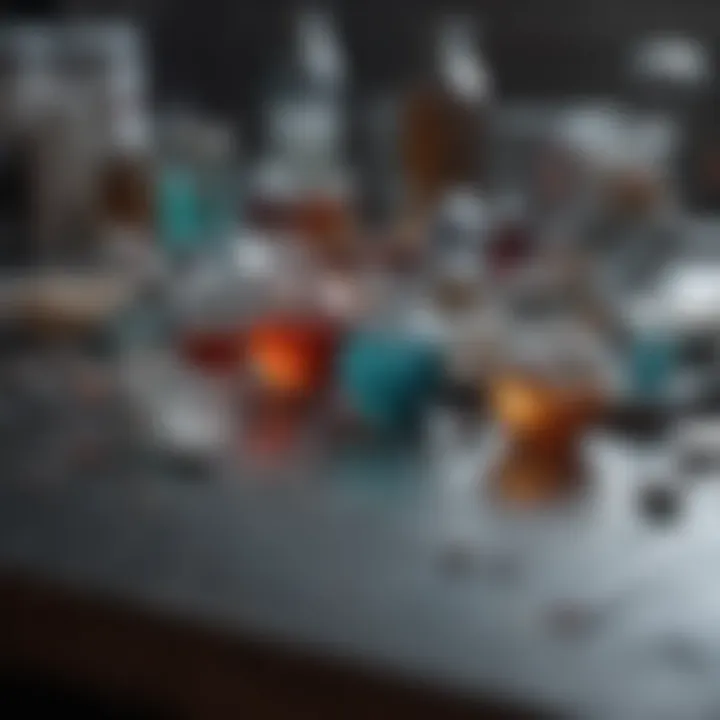
Intro
Modelling materials play a crucial role across various scientific domains. These materials are essential for simulating real-world phenomena and advancing research. Their understanding is not only vital for students and professionals in the field but also for anyone interested in the implications of modelling in science.
As the complexity of challenges increases in areas such as materials science, biology, and engineering, the demand for effective modelling materials grows. This article seeks to illuminate the landscape of modelling materials through an extensive analysis of their types, properties, applications, and future trends.
Research Overview
Summary of Key Findings
In this article, we explore the wide array of modelling materials available today. Key findings reveal that materials can be categorized based on their physical and chemical properties. For instance:
- Metals such as aluminum and copper are widely used due to their malleability and conductivity.
- Polymers, including silicone and polyethylene, offer versatility and adaptability.
- Ceramics are valued for their hardness and thermal stability.
Our findings indicate that each material type has distinct advantages depending on the application. Moreover, the environmental implications of these materials are becoming increasingly pertinent in research and development.
Background and Context
The study of modelling materials is underscored by a rich historical context. From early usages in scientific experiments to contemporary applications in advanced engineering, the evolution of these materials reflects technological advancements. The integration of modelling materials into research serves as a bridge between theoretical exploration and practical implementation. In many cases, they enable scientists to develop hypotheses, conduct experiments, and validate their results in an efficient manner.
Methodology
Experimental Design
The examination of modelling materials involves rigorous experimental design. The aim is to assess how different materials behave under various conditions. Factors such as temperature, pressure, and environmental exposure are critical in determining material effectiveness.
Data Collection Techniques
Collecting accurate data is essential for thorough analysis. Techniques such as:
- Spectroscopy for material identification
- X-ray diffraction for analyzing crystal structures
- Mechanical testing to gauge strength and durability
are commonly employed. Gathering this data allows scientists to create a well-rounded profile of each material, ensuring that their applications are based on solid evidence.
"In-depth understanding of modelling materials can lead to significant advancements in both academic and practical realms of science."
By systematically detailing materials’ properties and testing conditions, we can predict their behavior in various situations, further enhancing their application scope.
Culmination
In examining modelling materials, one gains insight into not just their physical characteristics but also their overarching implications in scientific inquiry. This exploration will systematically delve deeper into specific materials in the following sections, highlighting their significance in modern research and potential future developments.
Prologue to Modelling Materials
Modelling materials play a crucial role in the fields of science and engineering. Their versatility and functionality allow them to serve various purposes, from structural design to biomedical applications. Understanding these materials is essential for researchers and professionals alike, as it informs not only theoretical frameworks but also practical solutions to complex problems. This section delves into the multidimensional nature of modelling materials, aiming to illuminate their significance in modern scientific endeavors.
In studying modelling materials, one must consider several important elements. First, the classification of materials into natural, synthetic, and composite categories helps in understanding their fundamental differences. Each type has distinct properties and applications, making it pertinent to select the appropriate material for specific tasks. Secondly, the historical context of modelling materials reveals how advancements in technology have influenced their development and usage across time. This historical perspective can guide future innovational directions.
Definition of Modelling Materials
Modelling materials refer to various substances used in the creation of models, representations, or prototypes for testing and analysis. These include but are not limited to plastics, metals, ceramics, and biological materials. The choice of material often depends on the intended application and required characteristics, such as strength, durability, and ease of manipulation.
These materials can be used in a wide range of disciplines, making them integral in experimental design. For example, in engineering, modelling materials assist in simulating real-world conditions to optimize designs. In biomedical contexts, they contribute to the creation of prosthetics and other medical devices that require a high level of biocompatibility.
Historical Context
The use of modelling materials dates back centuries, with early civilizations employing clay and stone to create functional objects and artistic representations. The evolution of materials science dramatically changed the landscape of modelling materials. During the Industrial Revolution, advances in metalworking and the introduction of synthetic polymers expanded the range of available materials.
In the 20th century, innovations such as 3D printing spurred a remarkable shift in how modelling materials are approached and utilized. This technology allowed for the creation of complex geometries that were not feasible with traditional methods. Moreover, the rise of nanotechnology has opened up new possibilities for designing materials with enhanced properties, making them ideal for more specialized applications. Understanding this historical context is essential in appreciating the current state of modelling materials and their future trajectory.
Types of Modelling Materials
Understanding the various types of modelling materials is crucial for grasping their unique benefits and applications across different fields. Each type offers specific properties that cater to various needs, allowing researchers and professionals to select the most appropriate material for their projects. The distinction among these materials—natural, synthetic, and composite—highlights the complexity and versatility that characterizes the domain of modelling materials.
Natural Materials
Natural materials are those sourced from nature without any significant chemical alteration. Examples include clay, wood, and certain types of stone. These materials have been utilized for centuries in various forms of modelling, including art and architecture.
Benefits of Natural Materials:
- Sustainability: Many natural materials are renewable resources. Their extraction and use have a lower environmental impact compared to synthetic alternatives.
- Aesthetic Appeal: Natural materials often have unique textures and colors, which can enhance the visual aspects of a model.
- Thermal Properties: Materials like wood can provide good insulation, making them suitable for certain applications.
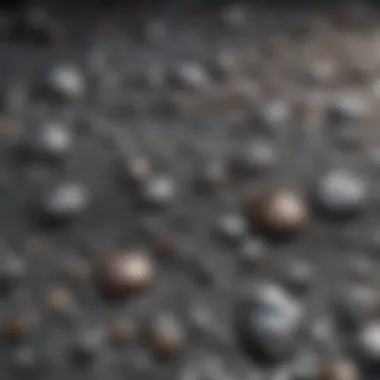
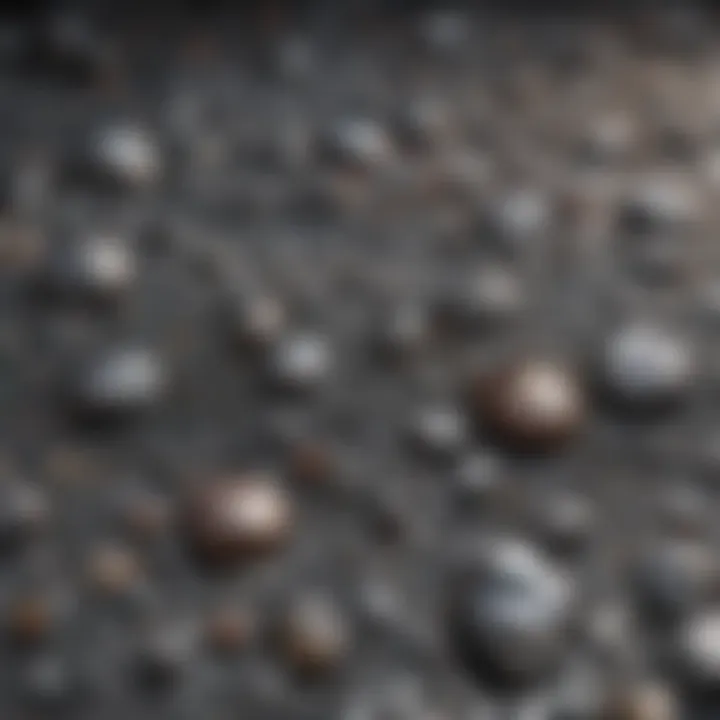
Considerations:
- Resource Availability: Depending on geographic location, sourcing natural materials might pose challenges.
- Degradation: Natural materials may be prone to decay, moisture absorption, or insect damage if not properly treated.
Synthetic Materials
Synthetic materials are engineered from chemical compounds, designed to exhibit specific properties not typically found in natural substances. Examples include plastics, polymers, and resins. These materials are often used in modern modelling applications due to their tailored characteristics.
Benefits of Synthetic Materials:
- Durability: Synthetic materials are typically more resistant to degradation and can withstand harsher conditions than natural options.
- Customization: They can be engineered to have desired properties such as flexibility, strength, or chemical resistance.
- Consistency: The manufacturing process allows for uniformity in material performance, which can be crucial for precision in modelling.
Considerations:
- Environmental Concerns: The production and disposal of synthetic materials can lead to pollution and waste issues.
- Cost: Often, synthetic materials can be more expensive due to production processes.
Composite Materials
Composite materials combine two or more distinct materials to create a final product that boasts superior properties. Common examples include fiberglass and carbon fiber. These materials are increasingly popular in engineering and scientific fields for their enhanced strength-to-weight ratios.
Benefits of Composite Materials:
- Enhanced Properties: The combination can result in a material that is stronger, lighter, or more resistant than its individual components.
- Versatility: They can be tailored for specific applications, making them suitable for a wide range of modelling needs.
- Reduced Maintenance: Many composites are resistant to moisture, corrosion, and temperature fluctuations.
Considerations:
- Complex Manufacturing: The production of composites can require specialized techniques, which may limit accessibility.
- Higher Initial Costs: While durable, composite materials can have a higher upfront cost compared to traditional modelling materials.
In summary, the choice of modelling materials impacts the efficiency and effectiveness of scientific and engineering applications. By exploring the strengths and weaknesses of natural, synthetic, and composite materials, professionals can make informed decisions that contribute to successful outcomes in their projects.
Properties of Modelling Materials
The properties of modelling materials play a crucial role in determining their suitability for various applications. These characteristics affect not only the performance but also the longevity and reliability of materials in different environments. Understanding these properties allows scientists and engineers to select the most appropriate material for specific tasks. The key properties generally include mechanical, thermal, and chemical characteristics.
Mechanical Properties
Strength
Strength is a fundamental property that indicates a material's ability to withstand external forces without deforming or breaking. Different modelling materials exhibit varying levels of strength. This property is vital for ensuring that structures or models can support loads and resist damage over time. A strong material reduces the need for additional support, making designs more efficient and cost-effective.
A key characteristic of strength is its measurement, typically in units of pressure such as Pascals or megapascals. This allows for consistent comparison across materials. Strong materials like metals are often favored in structural applications, but their weight can be a drawback. Lightweight materials, while generally weaker, can still be effective, depending on the application.
Advantages of choosing strong materials include increased safety and structural integrity. However, selecting a material solely based on strength might overlook other essential factors such as cost and ease of fabrication.
Elasticity
Elasticity refers to a material's ability to return to its original shape after being deformed. This property is significant in applications where flexibility is needed, such as elastomers used in seals and gaskets. Elasticity is characterized by the modulus of elasticity, which quantifies how much a material deforms under stress.
The usefulness of elastic materials lies in their ability to absorb shock and reduce vibrations. This characteristic is particularly beneficial in automotive and aerospace applications, where safety and performance are vital. However, excessive elasticity can lead to instability in structures, necessitating careful selection based on the intended use.
One unique feature is the hysteresis loss in elastic materials, which can waste energy through heat generation. This factor should be considered when using elastic materials in energy-efficient designs.
Durability
Durability is the property that measures a material's ability to resist wear, pressure, or damage over time. Durable materials are essential in harsh environments, where exposure to physical stress and chemical attacks is common. The durability of a material can drastically affect its lifespan and maintenance requirements.
A critical characteristic of durability is its relationship to other properties, such as strength and corrosion resistance. For example, metals like stainless steel are known for their durability because they resist rust and corrosion, making them popular in construction. While durable materials can initially have a higher cost, they often provide long-term savings due to lower maintenance needs.
One disadvantage of some highly durable materials is their potential rigidity, which can limit use in flexible applications.
Thermal Properties
Conductivity
Thermal conductivity measures a material's ability to conduct heat. Materials with high thermal conductivity, like metals, are essential in applications requiring efficient heat transfer, such as heat exchangers and thermal insulation. Understanding thermal conductivity assists engineers in selecting suitable materials for thermal management.
Key characteristics of conductors include how quickly they transfer heat relative to their volume. Increased conductivity can enhance operational efficiency in heating and cooling systems. However, high thermal conductivity may not always be desirable, such as in insulating applications where heat retention is needed.
The unique feature of thermal conductivity is its dependency on temperature; many materials’ conductivity changes at various temperatures, making it crucial to understand this aspect during design.
Thermal Expansion
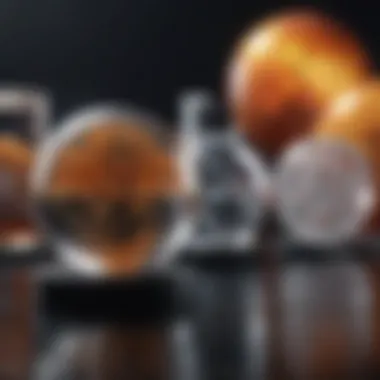
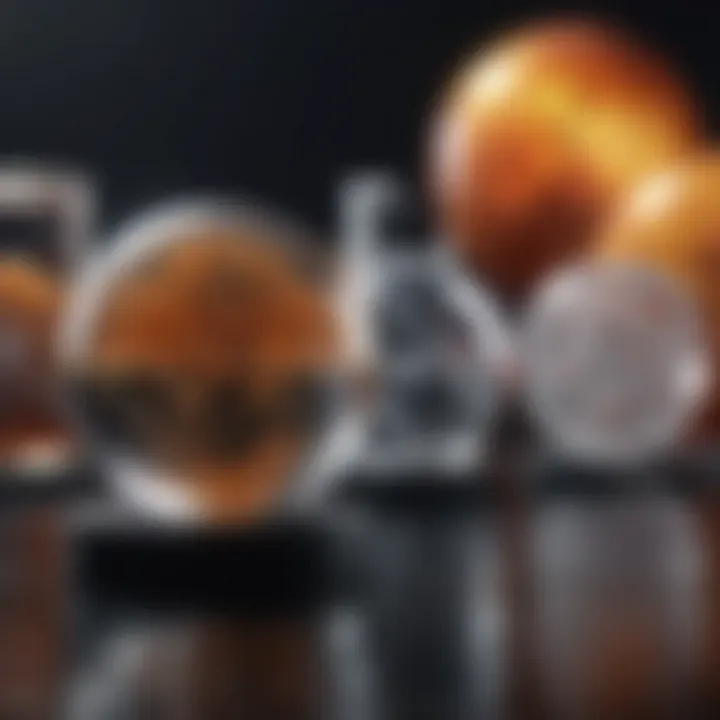
Thermal expansion describes how materials change in size or volume in response to temperature variations. This property is significant in applications where temperature fluctuations are common, such as machinery parts and electronic devices. Understanding thermal expansion helps prevent structural failures from thermal stresses.
A key characteristic of thermal expansion is its coefficient of expansion, which quantifies how much a material expands per degree of temperature change. This property is essential when materials with different expansion rates are joined, as they may induce stress and lead to failure.
One unique aspect of thermal expansion is its ability to be engineered to match expansion characteristics in composite materials, which is often necessary in advanced manufacturing processes.
Chemical Properties
Corrosion Resistance
Corrosion resistance is the ability of a material to withstand degradation due to chemical reactions, particularly oxidation and exposure to moisture. This property is crucial in environments where materials face harsh chemicals or cyclic exposure to water. Selecting materials with excellent corrosion resistance helps ensure longevity and safety in engineering projects.
A key characteristic of corrosion-resistant materials is the formation of protective layers, such as those found in stainless steel and aluminum. These layers prevent further degradation, prolonging the material’s life. However, selecting materials solely based on corrosion resistance may overlook other essential parameters, such as strength and cost.
One disadvantage of highly resistant materials can be their complexity to install or fabricate. That can lead to increased project timelines or costs, which should be carefully considered.
Reactivity
Reactivity indicates how a material interacts with other substances, particularly under environmental conditions. Understanding reactivity is vital in applications where materials come into contact with chemicals. The properties define a material's stability and inform safety considerations during handling and use.
A key characteristic of reactivity is its trends with environmental factors, which can significantly alter performance. Materials deemed as stable tend to be more desirable due to their predictability during application. Unstable materials may require additional containment measures to prevent accidents or material loss, adding complexity to project designs.
One unique factor is the reactivity of metals with acids, which can result in rapid degradation. Thus, careful planning and material selection are vital to ensure safe operations.
Applications of Modelling Materials
Modelling materials have extensive applications across various fields. Understanding these applications aids in appreciating their significance in both theoretical research and practical implementations. The use of modelling materials is critical in engineering, medicine, and environmental science. These fields rely on accurate representations to solve complex challenges and improve outcomes. Each application has unique benefits that highlight the versatile nature of these materials.
In Engineering
Structural Modelling
Structural modelling involves creating representations of physical structures to assess their viability. This application is crucial for safety and optimization in construction projects. Engineers use various modelling materials to simulate real-world conditions, helping them understand how structures will behave under stress. A key characteristic of structural modelling is its ability to predict material failure points. This predictive advantage makes it a popular choice, as it informs design modifications that enhance structural integrity. However, one disadvantage remains: the cost associated with high-quality materials which can discourage wider adoption.
Thermal Modelling
Thermal modelling focuses on heat transfer processes within materials. It plays a vital role in designing efficient thermal systems. Engineers utilize thermal modelling to simulate temperature distributions and identify the best insulation materials. The key characteristic of thermal modelling is its precise heat analysis, allowing for effective energy management in various applications. This benefit makes it an essential approach in disciplines like HVAC and automotive industries. On the downside, the complexity of thermal modelling can lead to longer project timelines, as many intricate variables must be considered.
In Medicine
Prosthetics
Prosthetics are artificial devices designed to replace lost limbs or body parts. They represent a remarkable application of modelling materials that significantly enhance the quality of life for users. A key aspect of prosthetics is their tailored design, which allows them to meet individual needs, improving functionality. This uniqueness is a major benefit that advances personal mobility and independence. However, a notable challenge is the ongoing maintenance and adaptation of prosthetics, which requires continual innovation and careful engineering.
Biocompatible Materials
Biocompatible materials are specially designed for medical applications. They must not induce significant immune responses. These materials are crucial in developing implants and devices that integrate safely with the human body. A significant advantage of biocompatible materials is their ability to promote healing and reduce the risk of infection. However, the unique feature of biocompatibility comes with challenges. The materials can be costly, limiting access for some patients and complicating the production processes.
In Environmental Science
Pollution Modelling
Pollution modelling is a key tool in understanding and addressing environmental issues. It involves simulating the dispersion and impact of pollutants in different ecosystems. A key characteristic of pollution modelling is its ability to provide insight into the effectiveness of mitigation strategies. These advantages make it a preferred choice for researchers aiming to develop sustainable solutions. Still, the main disadvantage is the reliance on accurate data. Inaccurate inputs can lead to misleading conclusions, affecting policy decisions and public health.
Habitat Restoration
Habitat restoration involves the process of returning degraded environments to a stable state. This application is crucial for biodiversity conservation. The unique feature of habitat restoration is its focus on long-term ecological balance, which benefits ecosystems over time. This approach is increasingly popular as awareness of ecological impacts grows. Nevertheless, restoration projects can often face challenges such as funding and time constraints, which may hinder effectiveness.
"The strategic application of modelling materials fosters innovation, supports sustainable practices, and resolves complex societal issues across multiple fields."
By exploring the applications of modelling materials, one gains a clearer picture of their multifaceted role in advancing science and enhancing societal welfare.
Technological Innovations in Modelling Materials
Technological innovations in modelling materials are reshaping various industries. These advancements enhance performance and open up new possibilities. Understanding these innovations is essential for comprehensively analyzing modelling materials. The integration of technology not only elevates the capabilities of existing materials but also facilitates the creation of entirely new material types. This section covers two significant areas: 3D printing technologies and nanotechnology applications.
3D Printing Technologies
3D printing has revolutionized the manufacturing landscape. It allows for precise control over material placement, which leads to remarkable structural integrity. The adaptability of this technology enables the production of complex geometries which were previously unattainable with traditional methods. Some key benefits include:
- Customization: Designs can be easily modified to fit specific needs, leading to tailored solutions.
- Reduced Waste: The additive nature of 3D printing minimizes material loss.
- Rapid Prototyping: Ideas can move from concept to physical models quickly, speeding up the R&D process.
- Material Variety: Multiple materials can be used in a single print, enhancing functionality.
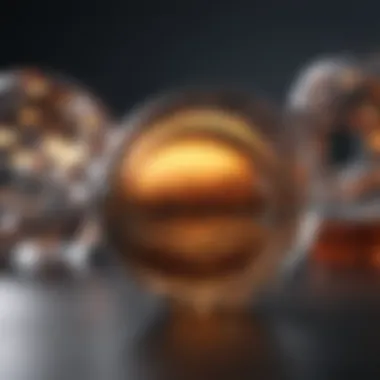
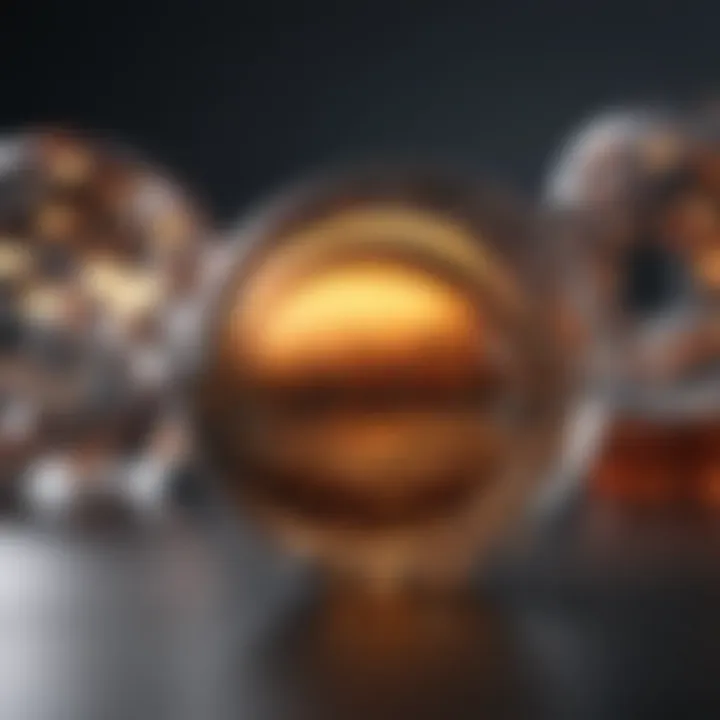
These benefits make 3D printing a favorite in various fields such as aerospace, architecture, and biomedical engineering.
"3D printing can produce parts with intricate designs that are otherwise impossible to create by traditional manufacturing methods."
Materials such as photopolymers, metal alloys, and thermoplastics have been optimized for use with 3D printing. As this technology evolves, new materials will likely emerge, continuously altering the landscape of modelling materials.
Nanotechnology Applications
Nanotechnology represents another frontier in modelling materials. By manipulating matter at the molecular or atomic level, materials can be engineered with extraordinary properties. This innovation unlocks potential that was once deemed impractical. Notable applications include:
- Enhanced Strength and Durability: Nanoparticles can significantly improve material toughness, making structures more resistant to stresses.
- Improved Conductivity: Materials can exhibit enhanced electrical or thermal conductivity, beneficial in electronics and energy applications.
- Self-Healing Materials: Innovations in nanotechnology lead to materials that can repair themselves when damaged, extending their lifespan and functionality.
The relevance of nanotechnology in modelling materials cannot be overstated. Its capacity to improve material performance fundamentally influences how materials are developed and utilized in application.
As research across both 3D printing and nanotechnology continues to progress, their impact on modelling materials offers exciting possibilities for the future. These technological advancements not only enhance existing applications but also create new avenues for exploration and innovation.
Challenges in the Use of Modelling Materials
The topic of challenges in the use of modelling materials is essential in understanding the practical limitations faced in various fields. These challenges can affect research outcomes, project costs, and the overall effectiveness of modelling applications. Recognizing the potential issues helps in formulating strategies to mitigate risks and improve the future use of these materials. Here, we will discuss material degradation and cost and accessibility, both of which significantly impact the selection and application of modelling materials.
Material Degradation
Material degradation refers to the deterioration of modelling materials over time due to environmental factors, mechanical stress, or chemical interactions. This issue poses a considerable challenge, especially in long-term applications, where reliability is critical. Common factors contributing to degradation include:
- Ultraviolet Light Exposure: Many synthetic materials become brittle or discolor when exposed to UV light over extended periods. This can compromise structural integrity.
- Moisture and Humidity: Variations in moisture levels can cause materials to swell or shrink. This not only affects the physical properties but also the overall functionality of models.
- Temperature Fluctuations: Extreme heat or cold can lead to thermal expansion or contraction, which may cause warping or cracking.
- Chemical Reactions: Some materials are prone to reactions with specific chemicals, altering their properties and reducing lifespan.
Addressing material degradation can involve several strategies, such as selecting more resilient materials, applying protective coatings, or implementing regulatory assessments to evaluate long-term performance.
"Understanding material degradation is crucial to extending the lifespan and functionality of modelling materials in various applications."
Cost and Accessibility
Cost and accessibility are pivotal challenges in the adoption of specific modelling materials. The initial investment for high-quality materials can be substantial, often discouraging researchers and educators from utilizing them. Factors affecting this challenge include:
- Material Sourcing: Not all modelling materials are readily available in all regions. Limited supply can drive up costs, making them less accessible for many users.
- Bulk Purchase Discounts: Smaller institutions or individual researchers may not have the capacity to purchase materials in bulk, reducing their negotiating power and leading to higher prices.
- Innovation and Research Funding: Many advanced materials require significant investments in research and development. Limited funding can diminish access to cutting-edge options that may offer better performance.
To navigate these challenges, collaboration between institutions, development of open-source platforms, and increased funding for research projects can enhance accessibility. Furthermore, exploring alternative materials that offer similar benefits at a lower cost could be essential in achieving broader application of modelling materials.
Future Trends in Modelling Materials
The landscape of modelling materials is evolving. Researchers and industry professionals are increasingly aware of how materials can align with global needs, especially concerning sustainability and adaptability. The importance of these future trends cannot be understated. They not only influence current practices but also set the stage for innovations that may redefine various applications in different fields. Understanding these trends allows stakeholders to better position themselves within sectors that rely on advanced materials. Moreover, it emphasizes the need for a systematic approach to research and development within this domain.
Sustainability Considerations
Sustainability is at the forefront of developing new modelling materials. The environmental impact of material production and disposal necessitates a shift towards more sustainable practices. The push for eco-friendly materials leads to innovations that focus on reducing carbon footprints and minimizing pollution.
- Biodegradable materials: These are designed to decompose naturally. Research is ongoing to engineer materials that break down efficiently without harming ecosystems.
- Recycled materials: Utilizing post-consumer products reduces waste. The development of high-performance materials from recycled sources has gained traction.
- Energy-efficient processes: Innovations in manufacturing can lower energy consumption. This includes using renewable energy sources and optimizing production methods to reduce waste.
Incorporating sustainable practices is vital, not only for the environment but also for future cost efficiency.
Adaptive Materials
Adaptive materials represent a new frontier in modelling. These materials can respond dynamically to environmental changes, offering significant advantages in various applications. The ability to change properties based on external stimuli can lead to enhanced functionality in multiple domains.
- Self-healing materials: Such materials can autonomously repair damage. This reduces the need for replacements and repairs, thus extending service life.
- Shape-shifting materials: These can change their shape in response to heat or stress. Engineering these materials could revolutionize sectors such as aerospace and automotive.
- Smart materials: Integrated technologies allow these materials to react to signals. In the healthcare field, smart bandages can monitor and adapt to changes in a patient’s condition.
As the demand for higher performance and efficiency grows, adaptive materials are likely to play a critical role in future development strategies.
"The future lies in materials that can adapt, respond, and heal themselves, ushering in an era of intelligent engineering."
Finale
The conclusion serves as a vital synthesis of the insights gathered throughout this analysis of modelling materials. It emphasizes the intricate relationship between various types of modelling materials and their applications across numerous scientific domains. The discussion highlights how these materials not only serve practical purposes but also bridge theoretical concepts with real-world scenarios.
One essential aspect of the conclusion revolves around the importance of understanding the properties of these materials. Mechanical, thermal, and chemical properties play crucial roles in determining the suitability of materials for specific applications. For instance, in engineering, selecting the right material can impact not only structural integrity but also long-term sustainability.
Furthermore, the conclusion addresses the ongoing technological innovations that continue to revolutionize the field of modelling materials. Developments such as 3D printing and the integration of nanotechnology open new avenues for creating more efficient and effective materials. Such advances are critical for meeting the evolving demands of different scientific sectors.
Another significant consideration outlined in the conclusion is the challenges that persist in the realm of modelling materials. Issues like material degradation and cost implications can hinder progress. By recognizing these challenges, researchers and practitioners can work towards solutions that facilitate better accessibility and longevity of materials used in various applications.
In summary, comprehending the vast landscape of modelling materials is essential for researchers, educators, and industry professionals alike. Not only does this knowledge enhance theoretical understanding, but it also informs practical applications that are crucial for innovation and effectiveness in multiple fields.
This article not only explores these dimensions but also sets the stage for future inquiries into the sustainability and adaptability of modelling materials. The future trends discussed signal a shift towards more conscientious choices in materials science, enabling a greater alignment with global sustainability goals.
The conclusion not only encapsulates the core themes explored but also underscores the ongoing relevance of modelling materials as society advances into a more high-tech and environmentally-conscious future.
In essence, the remarks made here advance the narrative of this article, paving the way for further exploration and understanding of modelling materials in the years to come.