Exploring DNA Fragmentation: Methods and Impact
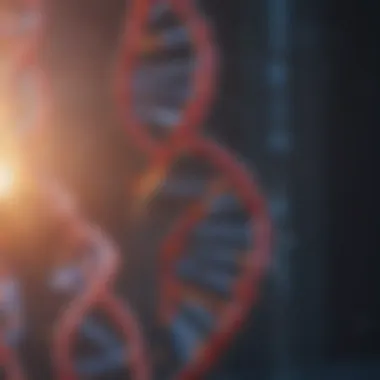
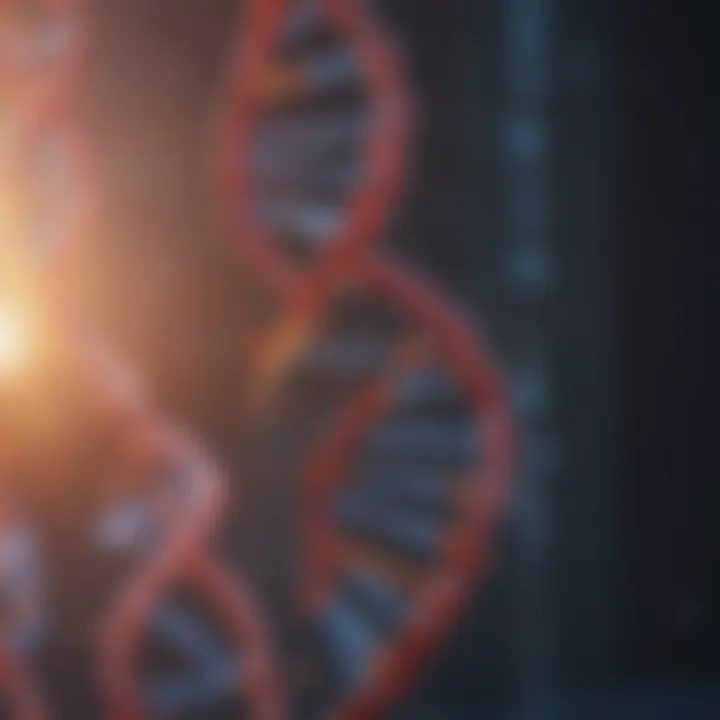
Intro
DNA fragmentation is a cornerstone of modern genetics, acting as a pivotal process in a wide array of scientific fields. From understanding genetic diseases to advancing biotechnological innovations, the cutting of DNA into fragments serves numerous purposes. The mechanisms behind this process involve intricate methodologies and specialized enzymes that enable researchers to manipulate genetic material precisely.
In recent years, the implications of DNA fragmentation have reached beyond the laboratories into ethical discussions, particularly regarding genetic engineering and gene therapy. This article sets out to explore the detailed processes involved in DNA fragmentation, examine the methodologies utilized, and evaluate the impact this practice has on current and future scientific endeavors.
Research Overview
Summary of Key Findings
Exploring the mechanisms involved in DNA fragmentation reveals several key findings:
- Enzymatic Actions: Specific enzymes, such as restriction endonucleases, play crucial roles in cutting DNA sequences, allowing researchers to isolate genes of interest.
- Techniques and Applications: Techniques like gel electrophoresis and PCR (Polymerase Chain Reaction) for analysis of fragmented DNA are critical in molecular biology.
- Ethical Considerations: Ethical implications, particularly in the context of gene editing, require careful consideration as they join ongoing debates in technology and society.
Background and Context
To appreciate the complexity of DNA fragmentation, it’s necessary to delve into its historical context. Techniques for DNA manipulation date back to the late 20th century with the advent of recombinant DNA technology. The capability to fragment DNA has allowed scientists to
- clone specific genes
- create genetically modified organisms
- diagnose genetic disorders
Understanding these historical foundations provides a backdrop for current practices and future advancements. By navigating the rich territory of molecular biology, we can perceive not just the mechanics of fragmenting DNA, but also how these techniques have evolved and stretched the limits of scientific inquiry.
Methodology
Experimental Design
In the realm of research, the experimental design for studying DNA fragmentation typically involves several integral steps:
- Selection of Samples: Choosing the right DNA samples, whether from bacteria, plants, or animals, is crucial for meaningful results.
- Fragmentation Techniques: Implementation of methods like sonication, chemical treatment, or enzymatic digestion directly influences the size and yield of DNA fragments.
- Analysis of Fragments: After fragmentation, techniques like agarose gel electrophoresis are often employed to visualize and assess the size distribution of DNA fragments.
Data Collection Techniques
Collecting data on fragmented DNA necessitates several techniques to ensure accuracy:
- Quantitative PCR: Allowing for the quantification of specific DNA regions post-fragmentation.
- Sequencing Technologies: Next-generation sequencing provides comprehensive data on the genetic information contained within the fragments.
As scientists continue to refine methodologies, the possibilities for applications across various disciplines greatly expand. Understanding the underlying mechanisms and their ethical ramifications is imperative for both the scientific community and society at large.
Understanding DNA Structure
Understanding the structure of DNA is like peering into the blueprint of life itself. In our quest to dissect the mechanisms behind cutting DNA into fragments, it is essential to grasp the very foundation of what DNA is composed of and how it functions. The intricate arrangement of nucleotides that make up DNA allows it to play a crucial role in various biological processes. This section seeks to elucidate the importance of that understanding, considering how each element interrelates to facilitate the innovative techniques that fragment DNA.
The Composition of DNA
Components of Nucleotides
Nucleotides are the building blocks of DNA, and they comprise three primary components: a sugar molecule, a phosphate group, and a nitrogenous base. Each type of nucleotide harbors a specific base: adenine, thymine, cytosine, or guanine. The key characteristic of nucleotides is their ability to form chains, which leads to the long strands we recognize as DNA. This method of construction renders nucleotides an advantageous choice when discussing DNA fragmentation, because their unique sequence determines the genetic information carried.
However, it's worthwhile to point out that while the inherent stability of these chains allows for reliable genetic fidelity, certain conditions can lead to mutations, which can create a significant disadvantage when working with genetic engineering.
Structure of the DNA Double Helix
A core feature of DNA is its double helix structure, which resembles a twisted ladder, where the sides are composed of alternating sugar and phosphate, while the rungs are formed by pairs of nitrogenous bases. This structure is pivotal to understanding how DNA fragments join, as it ensures that the bases pair according to specific rules. Consequently, it remains popular in discussions about DNA fragmentation as it facilitates comprehension of how fragments can recombine post-fragmentation.
The double helix's coiled form can be beneficial for protecting genetic material, yet its complexity may also pose difficulties when attempting to access or manipulate specific genes, which can complicate research.
Importance of Base Pairing
Base pairing is essential in keeping the DNA structure intact, as adenine pairs with thymine and cytosine pairs with guanine. This unique feature serves as a guide for DNA replication and repair, aiding in cellular processes. The importance of base pairing is highlighted in the context of DNA fragmentation; understanding base pairing can direct researchers in determining how DNA fragments can be reassembled correctly. This characteristic makes the knowledge of base pairing indispensable for any genetic manipulation.
Nevertheless, it is crucial to consider that any errors in base pairing during recombination processes can lead to significant genetic consequences, which can potentially derail an experiment or therapeutic approach.
Function of DNA in Cellular Processes
DNA Replication
DNA replication is the process that ensures genetic continuity from cell to cell. It involves unwinding the double helix and synthesizing a new strand complementary to each template strand. This process signifies the importance of DNA replication, as it provides the fundamental basis for cell division and the propagation of genetic information.
The hallmark of this function is its high fidelity—ensuring that the replicated strands closely mirror the original strands. However, the risk of inaccuracies, such as mutations during this process, poses a disadvantage in experiments involving DNA fragmentation. Such implications can potentially alter the final outcome of genetic manipulation efforts.
Transcription and Translation
Transcription and translation serve as processes through which the genetic instructions encoded in DNA are converted into functional proteins. Transcription involves synthesizing messenger RNA from the DNA template, leading to the expression of specific genes. Translation, on the other hand, reads this mRNA to assemble amino acids into proteins. This interplay emphasizes the importance of understanding these processes when discussing DNA fragmentation, as the point at which DNA is cut could affect gene expression significantly.
A benefits in this area is seen in synthetic biology where careful manipulation of these processes can lead to designing novel proteins. However, further complications emerge when fragments might not encode proteins adequately, leading to unexpected results.
Gene Regulation
Gene regulation plays a pivotal role in controlling which genes are expressed at any given time. This is achieved through complex networks of signals that determine gene activity. Understanding gene regulation is critical when investigating DNA fragmentation, particularly in scenarios where fragments may alter the regulation of genes nearby due to their insertion.
A unique feature of this regulation is its dynamic nature; decisions can shift due to environmental changes. This flexibility is beneficial for adaptation purposes but poses challenges when one considers the stability of genetic edits through fragmentation and subsequent insertion.
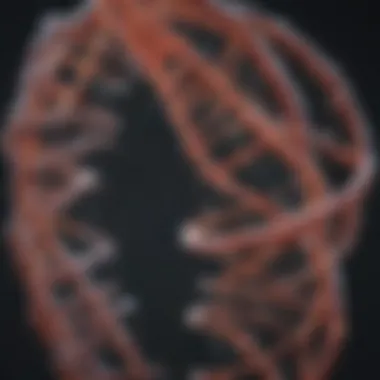
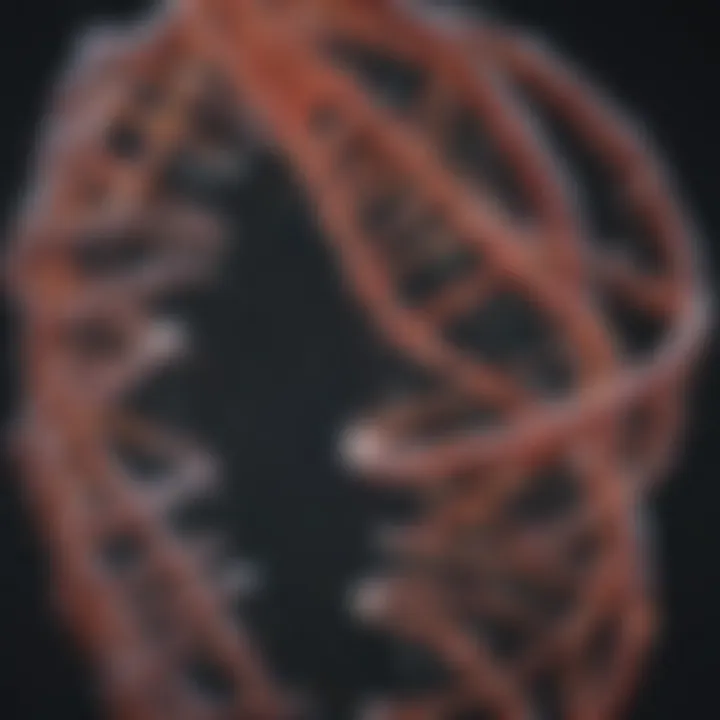
The interplay of these fundamental processes clarifies the intricate world of DNA, underlining its immense implications in the broader context of genetic research and technology. When researchers manipulate DNA, they tread the line between creative innovation and biological consequence. Understanding these mechanisms provides a sturdy foundation on which the whole field of genetic modification stands.
Techniques for Cutting DNA
Understanding the techniques for cutting DNA is crucial for a comprehensive grasp of genetic manipulation, molecular cloning, and even therapeutic applications. These methods serve as the backbone of genetic engineering, enabling researchers to dissect, analyze, and modify genetic material with precision. Each technique has distinct characteristics, advantages, and disadvantages, catering to specific research needs and experimental designs. As scientific inquiry evolves, the demand for effective and efficient DNA cutting techniques becomes ever more critical, shedding light on the pathways that lead to medical breakthroughs and innovations in biotechnology.
Restriction Enzymes
Definition and Discovery
Restriction enzymes, often referred to as restriction endonucleases, are proteins that slice DNA molecules at specific sequences. These enzymes were first discovered in the 1970s, particularly in studies involving bacteria, where they function as a defense mechanism against foreign DNA, such as viruses. Their ability to recognize unique palindromic sequences has made them an essential tool in molecular biology, particularly for cloning and genetic analysis.
The attraction of restriction enzymes lies in their specificity; they only target particular sequences, making them invaluable in ensuring precision during DNA manipulation. Moreover, their discovery opened the floodgates for techniques like recombinant DNA technology, which relies heavily on these enzymes to create novel genetic combinations.
Mechanism of Action
The mechanism of action of restriction enzymes is relatively straightforward but fascinating. Once the enzyme recognizes its specific target DNA sequence, it catalyzes a break in the phosphodiester backbone, creating distinct overhangs or blunt ends. This characteristic is paramount because it determines how DNA fragments can be joined together post-cutting, which is essential for cloning.
The beauty of restriction enzymes is in their dual role; they not only cut DNA but also allow for the precise reassembly of genetic material. Importantly, understanding how these enzymes function contributes significantly to the broader domain of gene editing and genetic engineering, as they serve as foundational tools in these applications.
Types of Restriction Enzymes
Restriction enzymes are classified into three main types: Type I, Type II, and Type III, with Type II being the most commonly employed in laboratories due to their straightforward cutting patterns and predictability. Type II enzymes, such as EcoRI and HindIII, recognize specific sequences and cleave at or near these sites without requiring ATP, which simplifies usage in various applications.
Each type offers unique advantages and limitations. For instance, while Type I enzymes can perform multiple functions, including methylation and cutting, they are less suitable for routine lab work due to their complexity. In contrast, Type II enzymes are a laboratory staple because of their reliable cutting patterns, making them highly beneficial for gene cloning and DNA analysis.
Endonucleases and Exonucleases
Differences Between Endonucleases and Exonucleases
Endonucleases and exonucleases are both critical in the realm of DNA fragmentation, but they serve different purposes. Endonucleases cut within the DNA strand, allowing for the removal or modification of specific regions. Conversely, exonucleases function by trimming nucleotides one by one from the ends of the DNA. This difference in action is what sets them apart fundamentally.
Endonucleases are especially useful in applications where precise cuts are mandatory, such as gene editing and recombination. Exonucleases, on the other hand, play a pivotal role in various DNA repair processes by nibbling away damaged nucleotides. Understanding these distinctions can guide researchers in selecting the proper enzymes suited for specific experimental conditions.
Applications in Genetic Engineering
Both endonucleases and exonucleases have found their niche in genetic engineering. Endonucleases are integral for strategies including CRISPR-Cas9, where scientists target, cut, and modify DNA at precise locations. This efficiency enables advancements in gene therapy, genetically modified organisms, and synthetic biology.
Exonucleases are equally important. In processes like DNA sequencing, they help ensure that only the desired strands remain, removing non-target regions for clearer analysis. The complementary roles of these classes of enzymes underline the versatility of DNA manipulation techniques, showcasing their utility across a broad array of applications in research.
Role in DNA Repair
The role of nucleases in DNA repair cannot be overlooked. Endonucleases are often triggered in response to DNA damage, cutting the affected sites and enabling subsequent repair mechanisms, such as homologous recombination or non-homologous end joining.
Exonucleases refine this repair process further by gradually trimming back damaged sections or correcting mismatches. Their contribution to maintaining genomic integrity makes them indispensable not only in laboratory settings but also in understanding cellular responses to DNA damage, which is vital in cancer research and therapeutic targets.
Physical Methods of DNA Fragmentation
Sonication
Sonication is a technique that utilizes ultrasound waves to induce mechanical fragmentation of DNA. When DNA solutions are subjected to high-frequency sound waves, the resultant shear forces cause the DNA strands to break at random sites. This method is particularly valuable when a large and uniform size distribution of fragments is needed for subsequent analyses, like sequencing.
One main advantage of sonication is its speed; it can produce DNA fragments almost instantaneously. However, controlling the fragment size can be tricky, as sonication often leads to a broad range of fragment lengths, which may not always be desirable for specific applications.
Mechanical Shearing
Mechanical shearing operates similarly to sonication, but it involves physical force to break DNA. Various devices, such as a French press or a rotor-stator homogenizer, are employed to shear DNA effectively. This technique is particularly useful when working with high-molecular-weight DNA, as it can generate desired fragment sizes more predictably compared to other mechanical methods.
While effective, mechanical shearing can introduce biases depending on the method and equipment used, leading to inconsistent results. Hence, good practice dictates optimizing protocols based on the particular requirements of downstream applications.
Laser Microdissection
Laser microdissection involves using a focused laser beam to precisely cut out specific DNA regions from a biological sample. This technique is particularly advantageous in isolating DNA from heterogeneous cell populations or tissue samples, where the target DNA may be buried among many others.
The key characteristic of this method is its high spatial resolution, allowing for nearly surgical precision in extracting DNA. While it offers unparalleled accuracy, the complexity and cost of the equipment can be a barrier for some laboratories.
Chemical Methods of DNA Fragmentation
Chemical Agents Used
Various chemical agents can induce fragmentation of DNA through reaction mechanisms that lead to strand breaks. Common agents include bleomycin and hydroxyl radicals, which target DNA through oxidative damage and can create defined breaks depending on the experimental conditions.
The specificity of chemical agents can be tailored based on the desired fragmentation pattern; however, handling these agents requires safety precautions due to their potential hazards. Understanding their mechanisms and appropriate usage is essential for effective application in laboratory settings.
Protocols and Procedures
Optimizing protocols for chemical fragmentation involves meticulous planning and execution. Specific concentrations of chemical agents, exposure times, and conditions like temperature can all influence the effectiveness of the reaction.
This precision in procedures is crucial because inconsistent methodologies can lead to varying results, complicating downstream applications. Following standardized procedures helps improve reproducibility across experiments, which is critical in scientific research.
Advantages and Disadvantages
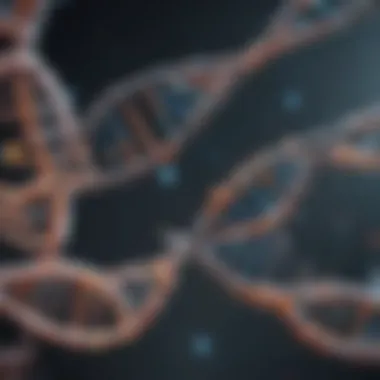
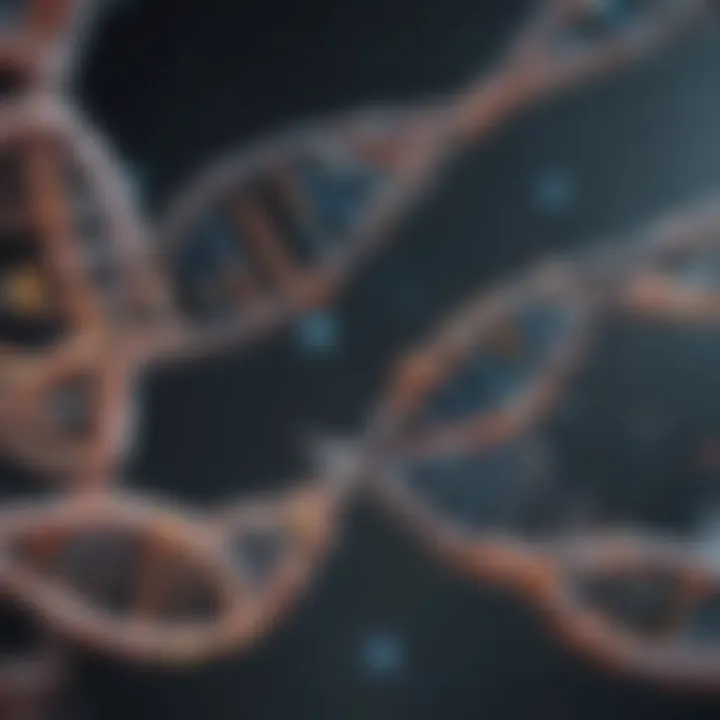
Chemical methods offer unique advantages, such as specificity in target sequences and controlled fragmentation, making them suitable for applications that require precise manipulation of DNA. However, the potential risks associated with using toxic chemical agents cannot be overlooked — they may introduce additional variables or unexpected reactions that confound results.
Researchers should weigh these pros and cons, considering their specific research goals and the potential implications of their chosen methods.
Applications of DNA Fragmentation
The ability to fragment DNA is not just a scientific curiosity; it plays a crucial role in numerous advanced applications across various fields. The implications of DNA fragmentation are vast and far-reaching, influencing molecular biology, genetic engineering, genomics, and clinical diagnostics. The techniques available for cutting DNA into fragments allow scientists to innovate and collect insights that could otherwise remain hidden. With this in mind, it is essential to understand the specific applications of DNA fragmentation, as they showcase the invaluable benefits and considerations necessary for further advancements in genetics.
Molecular Cloning
Principles of Molecular Cloning
Molecular cloning fundamentally revolves around the idea of creating copies of specific DNA sequences for manipulation and analysis. At its core, the process involves isolation of desired DNA fragments, followed by their insertion into vectors, enabling replication within a host organism. One key characteristic of molecular cloning is its precision: it allows researchers to target and amplify specific genes or genomic regions. This granularity makes it a beloved technique in research and gene studies, aiding in the creation of genetically modified organisms.
Unique to this approach is its ability to facilitate functional studies of genes, which are integral for understanding genetic traits or pathways. While highly effective, this method does come with its challenges, such as potential vector stability issues and the necessity for rigorous quality controls.
Vector Construction
Moving onto vector construction, this is pivotal in achieving successful molecular cloning. Vectors are essentially carriers that transport foreign DNA into host cells. A notable characteristic of vector construction is the diversity of vector systems available, ranging from plasmids to more complex viral vectors, to fit different research needs. For the article's context, vectors are considered a beneficial tool as they provide the necessary machinery for inserting DNA fragments within host cells, which can be beneficial for gene expression studies.
One unique feature of vector construction is the use of selectable markers, which allow researchers to easily identify successful transformations. However, one downside is that poorly designed vectors may lead to low transformation efficiencies or unintended consequences in host cells.
Insertion of DNA Fragments
The insertion of DNA fragments is a step that follows vector construction and is crucial for successful cloning outcomes. This process typically employs various methods including ligation, where DNA fragments are enzymatically joined. The primary characteristic of this process is that it forms stable recombinant DNA molecules, enabling further manipulation or expression. In the context of this article, insertion is regarded as a popular choice because it directly determines the efficiency of cloning and subsequent analyses.
A unique aspect of inserting DNA fragments is compatibility between the fragment and vector, which can heavily influence the success rate. There are often trade-offs, such as deciding between high-efficiency ligation methods and simpler, more straightforward protocols that may have lower yields.
Genetic Engineering and Synthetic Biology
CRISPR Technology
CRISPR technology represents a quantum leap in genetic engineering, allowing for precise modifications of the genome with a level of efficiency that traditional methods only dreamed of achieving. CRISPR stands out due to its ability to edit genes at specific locations, making it a darling of researchers aiming to create specific traits or understanding disease mechanisms. What's particularly intriguing is its versatility; it can be employed across a range of organisms, from bacteria to humans.
Its unique ability to deliver targeted edits is one of its most significant advantages, as it opens up a myriad of possibilities for gene therapies and disease treatment. However, the potential for off-target effects remains a concern, which researchers are continuously striving to mitigate.
Gene Therapy Applications
The applications of gene therapy are profoundly impactful as they can potentially cure genetic disorders by correcting defective genes. A significant aspect of gene therapy is its ability to directly target the underlying cause of diseases rather than merely alleviating symptoms. This therapeutic approach is especially beneficial for conditions like cystic fibrosis or certain types of inherited blindness.
One unique feature of gene therapy applications is the use of viral vectors to deliver therapeutic genes, as these vectors can efficiently penetrate target cells. However, the challenges of immune responses to these vectors present a noteworthy disadvantage in clinical settings, requiring researchers to adopt innovative strategies to overcome these barriers.
Designing Synthetic Genes
Synthetic gene design plays a vital role in expanding the boundaries of genetic engineering. The process involves constructing artificial genes that can produce desirable proteins or traits, thus offering a detailed toolkit for synthetic biology. The key characteristic of designing synthetic genes is the ability to fine-tune the genetic code to achieve specific outcomes. This has proven to be highly advantageous for fields like agriculture and pharmaceuticals.
An intriguing aspect is the possibility of incorporating non-natural amino acids to alter protein function. Yet, this can come with a downside, where the complexity of designing synthetic pathways may lead to unpredictable results, requiring thorough testing and optimization.
DNA Fragmentation in Genomics
Next-Generation Sequencing
Next-generation sequencing (NGS) has revolutionized the ability to read and analyze entire genomes. The process of DNA fragmentation is integral, as it allows for the generation of libraries containing short DNA fragments which can be sequenced simultaneously. One key characteristic of NGS is its throughput capability, enabling the sequencing of millions of fragments in a single run, making it an efficient choice in research.
A unique feature of NGS is its use of advanced computational algorithms to manage and interpret vast amounts of data collected during sequencing. Nevertheless, the high costs associated with sequencing platforms can be a limiting factor for smaller labs aiming to engage in genomic research.
Whole Genome Analysis
Whole genome analysis is a comprehensive approach to understanding the complete genetic makeup of an organism. The fragmentation of DNA allows for the detailed mapping and annotating of genomes, thus contributing to even deeper insights into genetics. This methodology is advantageous because it enables researchers to identify genetic variations that may have functional implications and understand evolutionary relationships.
A notable feature is the ability to detect structural variations within genomes, which can inform on genetic diseases. However, the challenge remains that the complexity of analyzing and interpreting large datasets can sometimes lead to inconclusive findings.
Comparative Genomics
Comparative genomics examines the similarities and differences in genomics across different species, offering profound insights into evolutionary biology. DNA fragmentation is crucial here, as it facilitates the comparison of genomic regions efficiently. A key characteristic is the ability to uncover conserved and diverged sequences, which can help in understanding speciation processes.
One unique feature is the application of bioinformatics tools that assist researchers in visualizing complex data. While comparative genomics illuminates many aspects of evolutionary biology, the interpretation of vast datasets requires a critical eye, as results can sometimes be misleading without proper contextual information.
Applications in Clinical Diagnostics
Detection of Genetic Disorders
The detection of genetic disorders has been transformed by advances in DNA fragmentation methodologies. By analyzing specific DNA fragments, clinicians can pinpoint mutations responsible for various hereditary conditions. A prominent aspect of this application is its ability to facilitate early diagnosis, which is crucial for implementing timely treatments.
A unique feature lies in the precision of targeted sequencing methods that focus on clinically relevant genes, increasing the chances of finding mutations. One downside, however, is that the need for expert interpretation of results can lead to delays in patient care if not efficiently managed.
Use in Personalized Medicine
Personalized medicine relies heavily on DNA analysis to tailor treatments to individual patients based on their genetic profile. The fragmentation of DNA aids in creating comprehensive profiles that inform clinical decisions. Its key characteristic is the alignment of treatments with a patient’s genetic makeup, which increases efficacy and reduces adverse reactions.
The uniqueness of this application is its potential to revolutionize how we approach treatment modalities. However, the challenge is ensuring that the necessary infrastructure and expertise are in place to handle the intricacies of personalized medicine effectively.
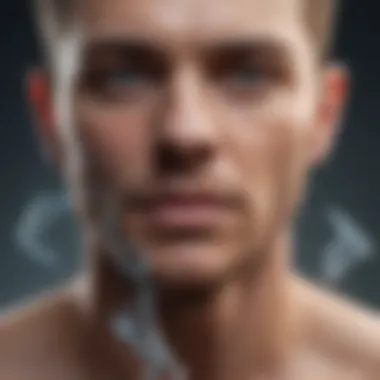
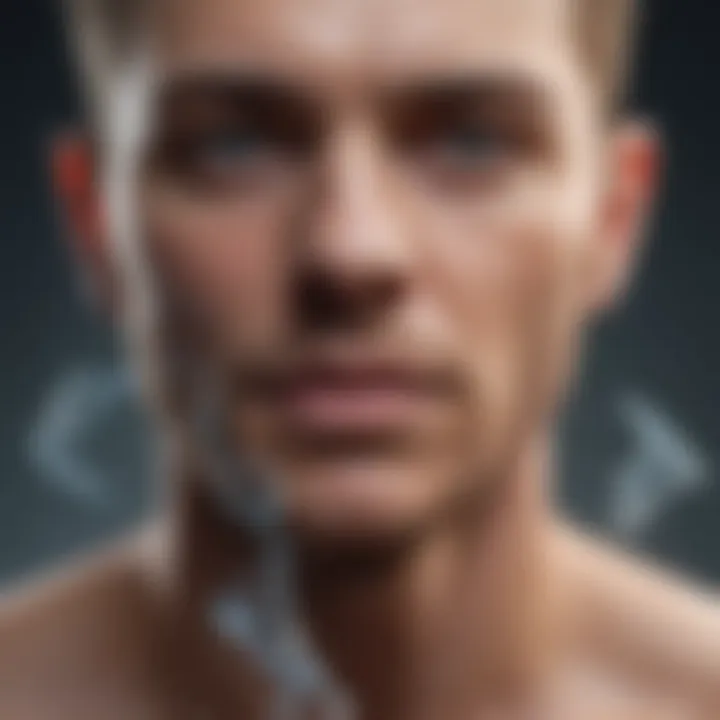
Role in Cancer Research
In cancer research, analyzing fragmented DNA from tumor samples provides insights into the genetic alterations that contribute to tumorigenesis. One specific aspect is the ability to identify biomarkers for early detection and prognosis, enhancing the options for targeted therapies.
A significant characteristic here is the rapid pace of discovery in uncovering novel targets for cancer treatment, making it a highly beneficial field for this article. A unique aspect is the integration of data from fragmented tumor DNA with other omics data for a holistic understanding of cancer biology. Yet, ongoing challenges such as tumor heterogeneity can complicate interpretations and require continuous research focus.
Ethical Considerations Surrounding DNA Fragmentation
Understanding the ethical landscape of DNA fragmentation is crucial as it delves into questions of legality, morality, and societal impact. The ability to manipulate genetic material raises important issues about consent, ownership, and the societal boundaries of genetic research. As scientists explore the potentials and implications of DNA fragmentation, it is fundamental to navigate these ethical considerations properly. In this section, we will amplify the conversation surrounding these topics, helping to clarify both the benefits and the challenges that accompany advances in genetic technologies.
Bioethics in Genetic Research
Consent and Ownership of Genetic Material
The conversation about consent and ownership of genetic material is at the forefront of bioethics. In an age where genetic information is invaluable, determining who has a right to this data is paramount. Consent must be informed, and individuals must have a clear understanding of how their genetic material will be used. The key characteristic here is autonomy—the individual’s right to make decisions about their own body.
This aspect is beneficial for the article as it stresses the importance of transparency in genetic research. Individuals' rights are non-negotiable, and violations can lead to mistrust, which hinders scientific progress. A unique feature of this topic is the emerging frameworks aimed at standardizing consent processes, which can either simplify or complicate data collection efforts depending on how they are implemented.
Potential for Genetic Discrimination
The risk of genetic discrimination poses a serious concern. As data on genes become more available, the potential for misuse of this information by employers or insurers looms large. The key characteristic of this issue is the intersection between genetic information and privacy rights, which creates a complex web of ethical dilemmas.
Identifying these risks is essential as we formulate guidelines for DNA research. Current laws exist to protect individuals from genetic discrimination, making this a timely topic for discussion. However, it’s not without challenges; enforcement of such laws tends to vary by jurisdiction. This inconsistency can lead to individuals being unfairly treated based on their genetic predispositions, highlighting a disadvantage in current frameworks.
Implications of Genetic Modification
The implications of genetic modification present both exciting possibilities and unsettling questions. Altering an organism's DNA can lead to breakthroughs in medicine and agriculture, but these advancements come with their own ethical baggage. The key characteristic here involves the unexpected consequences that might arise from altering natural processes.
While genetic modification can offer significant benefits, such as disease resistance in crops or new therapies for genetic disorders, it could also lead to potential ecological disruptions or health risks. Overall, a unique feature of this field is the ever-evolving dialogue between innovation, regulation, and public perception. Balancing these elements is crucial as science progresses.
Regulatory Frameworks
International Guidelines
When discussing international guidelines, it’s clear that they serve to unify the myriad of approaches to genetic research across borders. Such guidelines aim to establish a baseline for ethical considerations and promote responsible research practices. The key characteristic here is consistency in governance, which is essential as biotechnology becomes increasingly globalized.
The benefit is clear—having a uniform set of protocols helps to mitigate risks associated with genetic research. A unique aspect of international guidelines is their adaptability, allowing regions to integrate local regulations while still adhering to broader principles. However, some might argue that adherence to these guidelines can stifle innovation in regions that may need more lenient regulations to thrive.
National Regulations
National regulations significantly influence how genetic research is conducted domestically. These regulations often reflect cultural attitudes towards genetic research, highlighting the variability in ethical stances across different societies. The key characteristic of national regulations is that they are often reactive rather than proactive, shaping the landscape based on past events or public sentiment.
In this context, national regulations can serve as both a protective measure and a potential hindrance. While they ensure that ethical standards are upheld, they can also limit the scope of research and development. A unique advantage is that they allow for tailored rules that address specific societal needs and concerns, although this can lead to a patchwork of laws that complicates international collaboration.
Compliance and Oversight
Compliance and oversight are central to ensuring that genetic research adheres to ethical standards. These mechanisms help to regulate the practices of those conducting DNA fragmentation and related studies, instilling a sense of accountability. The key characteristic here is the establishment of bodies responsible for monitoring and enforcing regulations, which is fundamental for public trust in science.
The benefit of strong compliance frameworks is the reassurance they provide the public that ethical guidelines are being followed. However, complexities can arise when institutions have differing levels of resources and commitment to oversight. A unique feature of effective compliance is the engagement of various stakeholders, which can enhance the effectiveness of regulations while also fostering a culture of responsibility in genetic research.
Future Directions in DNA Fragmentation Research
As the field of genetic research continues to evolve at a staggering pace, the future directions in DNA fragmentation stand as a vital aspect shaping its trajectory. Understanding the mechanisms behind DNA fragmentation today can offer tantalizing glimpses into what lies ahead. Not only does it enable researchers to enhance current methodologies, but it also brings about innovative applications that tap into the potential of biotechnology. Hence, a deeper insight into future directions reveals its significance in paving the way for breakthroughs and enhancing the efficacy of genetic applications.
Advances in Techniques
Development of Next-Generation Enzymes
The surge in genomic studies has propelled the demand for more refined DNA manipulation tools. Next-generation enzymes are one area seeing a lot of activity. These enzymes, developed through advanced protein engineering techniques, exhibit enhanced properties like specificity and efficiency in cutting DNA. Consequently, they minimize off-target effects, which translates into more reliable research outcomes. For instance, enzymes like CRISPR-associated endonucleases have gained immense popularity due to their precise targeting and ease of use.
However, the field is still progressing, as researchers explore ways to improve enzyme performance in variable temperatures and conditions. One unique feature of these next-generation enzymes is their capability to work on a broader range of DNA sequences. This adaptability is paramount in genetic engineering as it allows for manipulation across diverse organisms.
New Physical Fragmentation Methods
Physical methods of DNA fragmentation are also moving forward. The development of new physical fragmentation methods leverages technology like microfluidics and acoustic waves to achieve precise fragmentation. This crucial aspect allows for a higher throughput, meaning more samples can be processed in less time.
What's appealing about these methods is their ability to produce consistent fragment sizes that suit specific applications, be it sequencing or cloning. However, one potential drawback is the cost of implementation. Setting up high-tech equipment can be a budgetary concern for many labs, particularly smaller ones.
Integration of Artificial Intelligence
Artificial intelligence (AI) is another game-changer in DNA fragmentation research. The integration of AI into genetic engineering can revolutionize how we understand and utilize DNA fragmentation. For example, machine learning algorithms can analyze vast datasets to optimize cutting strategies and predict outcomes more effectively than traditional methods. This approach not only saves time but also provides valuable insights that can enhance precision in experiments.
Despite its potential, AI in this domain must be approached with caution. Issues related to data privacy and ethical concerns about AI decisions in gene editing pose significant challenges. Nonetheless, the prospects of AI applying predictive analyses to tailor DNA fragmentation approaches is an exciting frontier in research.
Broader Applications in Biotechnology
Emerging Therapeutic Approaches
In the realm of biotechnology, emerging therapeutic approaches are rapidly evolving. As researchers explore new ways to harness DNA fragmentation, the field opens up novel avenues for therapy development. Genetic therapies utilizing tailored DNA fragments can offer more personalized treatment options for various genetic disorders. The adaptability of fragmented DNA, specifically designed to target specific genes, underscores its role in this context. However, navigating the regulatory landscape remains a challenge that could influence the pace of these innovations.
Enhancements in Agricultural Biotechnology
As agriculture faces tremendous pressure to increase yield and sustainability, advances in agricultural biotechnology derived from DNA fragmentation techniques stand as a beacon of hope. Techniques that allow for the precise manipulation of plant genomes enable crops to become more resilient to stressors such as climate change and pests. This approach not only enhances productivity but also aligns with sustainable practices. However, the public perception of genetically modified organisms (GMOs) can pose hurdles, necessitating effective communication and education efforts.
Impact on Conservation Biology
Lastly, the impact on conservation biology cannot be overlooked. Techniques being developed through DNA fragmentation play a pivotal role in preserving endangered species. For instance, these methods can aid in assessing genetic diversity by allowing researchers to analyze fragments from a variety of individuals. This genetic insight can inform conservation strategies and aid in species recovery efforts. However, caution is warranted as the reliance on cutting-edge technologies must balance ecological considerations to avoid unintended consequences.
In summary, the future of DNA fragmentation research is replete with potential and opportunities. From revolutionary techniques to broader applications in biotechnology, each step forward promises significant advancements. However, with every innovation, comes the responsibility to tread carefully, ensuring ethical implications are addressed while pursuing groundbreaking research.