Fluorophore Labeling of Proteins: Techniques and Applications
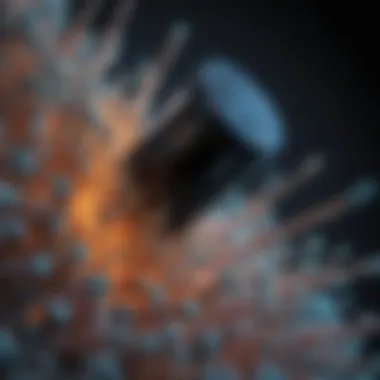
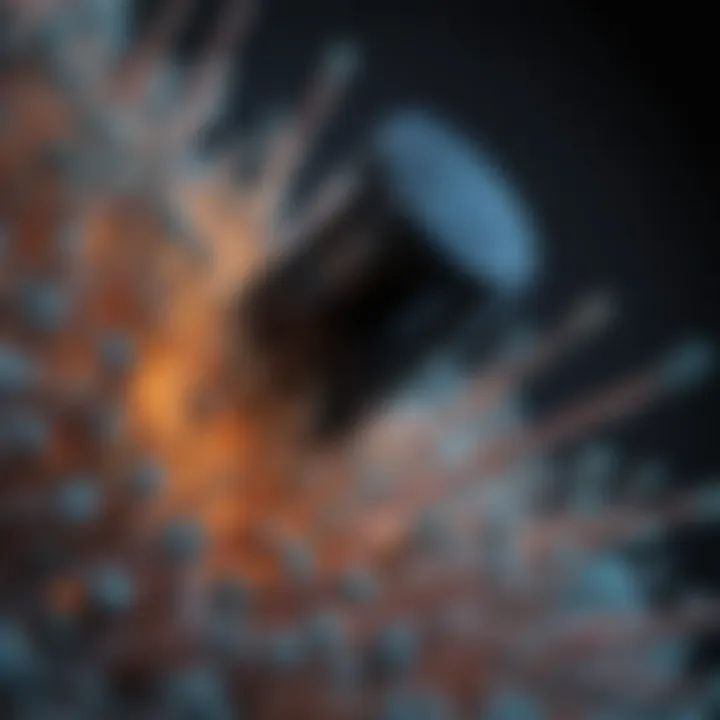
Intro
Fluorophore labeling of proteins stands as a cornerstone in contemporary biology. It allows scientists to tag proteins with fluorescent dyes, thereby enabling real-time visualization of cellular processes. This method is indispensable for deciphering the intricacies of protein interactions and behavior within various biological contexts. As researchers strive to unveil the mysteries of life at the molecular level, understanding how to utilize and implement fluorophore labeling effectively becomes paramount.
One key aspect of this technique is the ability to visualize protein dynamics in living organisms. This capability transforms data that was once static into a dynamic narrative, providing insights into protein location, movement, and function in situ.
This article aims to provide an extensive examination of fluorophore labeling, covering essential methodologies, key considerations for selecting appropriate fluorophores, and interpreting results effectively.
Research Overview
Summary of Key Findings
The studies surrounding fluorophore labeling reveal that not all fluorescent dyes are created equal. Factors such as excitation/emission spectra, photostability, and brightness must be taken into account when selecting a fluorophore. Researchers have demonstrated that careful optimization of labeling protocols can significantly improve data quality and reproducibility. Moreover, case studies highlight how fluorophore labeling has propelled advancements in areas like diagnostics and drug development.
Background and Context
Historically, the visualization of proteins posed significant challenges. Traditional microscopy methods provided limited insight, primarily due to resolution constraints. Fluorophore labeling, developed in the late 20th century, revolutionized this field. By using various fluorescence techniques, scientists can now observe proteins in real time, paving the way for advancements in cell biology and biochemistry. As scientific inquiry increases, the relevance of fluorophore techniques in understanding complex biological systems continues to grow.
"Fluorescence has brought a new dimension to our understanding of biology, illuminating pathways previously cast in shadow."
Understanding Fluorophores
Fluorophores, those light-emitting molecules, are at the heart of protein labeling techniques, serving as vital tools in microscopy and other imaging methods. Understanding fluorophores is crucial because they enable researchers to visualize the structure, dynamics, and interactions of proteins in real-time. The significance of this knowledge can't be overstated, as it lays the groundwork for numerous applications, from basic research to clinical diagnostics. This section aims to clarify what fluorophores are, how they function, and the different types available, thereby providing a solid foundation for the discussion on protein labeling.
Defining Fluorophores
Fluorophores are chemicals that can absorb light at specific wavelengths and then emit it at longer wavelengths. This property of absorbing and re-emitting light is what makes them highly valuable in various imaging applications. Essentially, fluorophores attach to proteins or other biomolecules, allowing scientists to monitor biological processes with remarkable precision. In practical terms, think of a fluorophore as a small beacon that can light up a target of interest within a complex biological system.
Mechanism of Fluorescence
The functioning of fluorophores revolves around the principle of fluorescence. When a fluorophore absorbs light, it gets excited to a higher energy state. Almost instantaneously, it returns to its ground state, releasing energy in the form of light. This process happens in a fraction of a second, creating a glow that researchers can track using sophisticated imaging systems. Essentially, the brightness and wavelength of this emitted light can provide insights regarding the nature and behavior of the labeled proteins.
Types of Fluorophores
Understanding the various types of fluorophores is essential because each has unique characteristics that might make it more suitable for specific applications. Below, we will delve into three primary categories of fluorophores: organic dyes, quantum dots, and fluorescent proteins.
Organic Dyes
Organic dyes are perhaps the most traditionally used fluorophores in laboratories. They're small, chemically synthesized compounds, and they better known for their vibrant colors.
- Key Characteristic: They generally offer a broad range of colors and are readily available.
- Benefits: Organic dyes are often favored because they are relatively inexpensive and easy to utilize.
- Unique Feature: One of their unique traits is their high molar absorptivity, which can lead to strong fluorescence intensity.
- Advantages/Disadvantages: However, they do have their downsides. For instance, many organic dyes are prone to photobleaching—losing their fluorescence after prolonged exposure to light, which can limit their effectiveness in long-term imaging studies.
Quantum Dots
Quantum dots represent a newer class of fluorophores known for their size-dependent optical properties. These semiconductor nanoparticles possess a unique behavior where their fluorescent properties can change with their size.
- Key Characteristic: They can emit emitted light at specific wavelengths depending on their size and material composition.
- Benefits: The durability and broad excitation range make quantum dots a compelling choice for fluorescent labeling, particularly in multi-color applications.
- Unique Feature: Their unique photostability is a significant advantage over organic dyes, allowing them to maintain brightness over extended periods.
- Advantages/Disadvantages: Yet, the relatively high cost and potential for toxicity raise concerns that need to be addressed when considering their use in biological settings.
Fluorescent Proteins
Fluorescent proteins, derived from organisms like jellyfish, have gained immense popularity due to their ability to tag proteins naturally in living cells.
- Key Characteristic: Unlike synthetic dyes, these proteins are genetically encoded, allowing them to be expressed within cells.
- Benefits: This property makes them invaluable because they can label proteins in situ without the need for additional chemical modifications.
- Unique Feature: The fact that they can be engineered to emit a variety of colors is a game-changer, enabling advanced imaging applications.
- Advantages/Disadvantages: On the flip side, their expression can sometimes be inconsistent, and the potential for cellular toxicity must be taken into consideration, which could impact experimental outcomes.
In summary, understanding fluorophores, their behavioral mechanisms, and the various types available is indispensable for effectively utilizing them in protein labeling applications. As we move forward, these fundamentals will serve as a basis for discussing advanced techniques and their applications in research.
Principles of Protein Labeling
Understanding the principles of protein labeling is essential in harnessing the full potential of fluorophore technologies. Labeling proteins with fluorophores allows researchers to track and visualize biomolecular processes in real time. By grasping the foundational aspects of this procedure, scientists can make informed decisions about labeling strategies that suit their experimental needs.
It's not just about sticking a bright tag onto a protein; it’s about understanding how that tag influences the protein's behavior, structure, and function. This principle serves not just as a stepping stone, but as a bedrock for the entire field of protein research.
Protein Structure and Function
The annals of biology are filled with stories of proteins, molecules that do heavy lifting in almost every biological process. To label them effectively, one must first appreciate that their structure is intricately connected to their function. Proteins are made up of amino acids that fold into complex shapes, with each shape determining the protein's job within the cell.
For instance, enzymes have active sites formed by specific arrangements of amino acids, while structures like antibodies are dependent on their unique conformation to recognize specific antigens. Because fluorophores bind to specific amino acids, labeling can influence protein stability and, ultimately, activity.
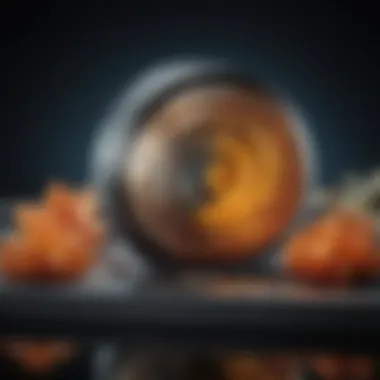
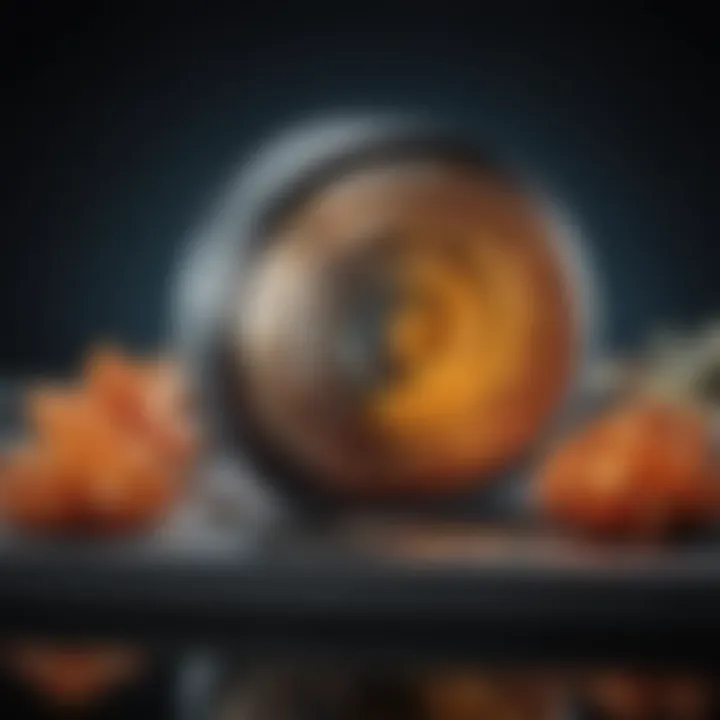
Labeling Concepts
When diving into the world of protein labeling, two primary concepts come to the fore: site-specific and non-specific labeling. Each type has its own set of characteristics that determine its application and relevance in research.
Site-Specific Labeling
Site-specific labeling shines as a preferred approach, especially because it minimizes perturbation to the protein's function. This method ensures that fluorophores attach to predetermined sites, often targeting specific residues like cysteine or lysine.
- Key Characteristic: It enhances specificity, allowing precise tracking of labeled proteins within different cellular contexts.
- Popularity: Site-specific labeling is often regarded as the gold standard due to its ability to preserve the protein’s native behavior.
- Unique Features: The use of recombinant technology or chemical strategies allows the incorporation of fluorophores without impacting critical functional sites.
While widely beneficial, site-specific labeling can also be complex as it often requires additional steps, including mutagenesis or the use of special linkers, which may complicate experiments.
Non-Specific Labeling
Contrasting with site-specific methods, non-specific labeling offers its own advantages. It often employs techniques like fluorescence isothiocyanate (FITC) or Alexa Fluor dyes that bind to various amino acids indiscriminately.
- Key Characteristic: Non-specific labeling provides a straightforward approach, allowing for quick and wider binding.
- Popularity: In some contexts, this method is favored for its ease of application, especially in high-throughput settings where time is of the essence.
- Unique Features: Though simpler, this method can lead to labeling of multiple sites and potentially filthier results, making it harder to trace specific proteins under experimental conditions.
While non-specific labeling is easy to implement, its lack of specificity might lead to complications in data interpretation. Overall, understanding these two concepts—site-specific and non-specific labeling—equips researchers with essential knowledge to choose the appropriate technique based on their specific research goals.
"The choice between site-specific and non-specific labeling techniques can shape the experimental landscape. Each method presents its own set of challenges and advantages, influencing the trajectory of research outcomes."
Together, these principles form the backbone of fluorophore labeling, where a clear grasp of protein structure and the different labeling concepts can enhance experimental design and application in biological research.
Labeling Techniques
Labeling techniques are at the heart of fluorophore labeling, serving as the backbone for how proteins can be visualized and studied in various contexts. Understanding these techniques is crucial for anyone diving into protein research, as they offer unique ways to uncover the intricacies of protein behavior within cells and tissues. With each technique presenting distinct advantages and challenges, mastering them is essential for achieving reliable and reproducible results.
Covalent Labeling
Covalent labeling involves creating a stable bond between a fluorophore and a specific amino acid within a protein. This method ensures that the label has a permanent attachment, which is essential for long-term studies. Covalent labeling typically involves reactive groups such as isothiocyanates or maleimides that interact with functional side chains like amino or sulfhydryl groups. Since this method offers high specificity, the labeling can reflect the precise localization and dynamics of the protein in question.
However, users must approach covalent labeling with a keen eye on potential complications. For example, modifications at critical sites of the protein might alter its natural function. Researchers should also consider factors such as solvent conditions, pH, and temperature, as these can significantly influence the efficiency of the reaction. Careful optimization of labeling conditions ensures minimal disruption to the protein's structure while maintaining functionality.
Non-Covalent Labeling
Non-covalent labeling, on the other hand, is less about direct chemical bonds and more about interactions. This technique often employs affinity tags or specific binding interactions that do not alter the protein structure. Examples include using biotinylated probes that bind to streptavidin-fluorophore conjugates, which permit straightforward visualization of the protein.
The ease of application with non-covalent labeling makes it an appealing choice, especially in live-cell imaging, as the fluorophore can be easily removed or changed without affecting the target protein. However, this flexibility can come at a cost; often the binding is less stable than covalent bonds, leading to concerns about background noise and the protein's behavior under varied conditions. The balance of specificity and stability needs careful consideration when selecting this route.
Genetically Encoded Fluorophores
Genetically encoded fluorophores represent a recent yet rapidly growing area in protein labeling. This method involves incorporating the genetic code of a fluorescent protein directly into the target organism or cell line. Proteins like Green Fluorescent Protein (GFP) and its derivatives have become staples in the field due to their robust and bright fluorescence properties.
One of the key advantages of genetically encoded fluorophores lies in their ability to be expressed within living organisms, allowing researchers to visualize protein dynamics in real-time. This has opened doors to new insights into cellular mechanisms, especially in context of developmental biology or disease progression.
Despite the clear upsides, this approach also presents challenges. Notably, the time needed for expression and subsequent maturation can be a concern. Moreover, the folding and functionality of the protein must be verified post-expression to ensure that the fluorescence truly correlates with the protein's presence and activity.
In all cases, the selection of the appropriate labeling technique should align with the research goals, considering both the desired outcomes and the inherent limitations of each method.
By examining these techniques, researchers can better understand how to design experiments that effectively utilize fluorophore labeling, achieving deeper insights into the roles and interactions of proteins in their native contexts.
Optimization of Labeling Protocols
Optimizing the labeling protocols of proteins with fluorophores is like tuning a finely crafted instrument before a performance. Just a few adjustments can make the difference between a dazzling display of fluorescence and a lackluster presentation. The essence of successful protein labeling lies in careful consideration of various factors that influence both the efficiency and accuracy of the results.
When embarking on fluorophore labeling, one must prioritize not just the selection of fluorophores but also the entire protocol surrounding the labeling process. This includes the conditions under which the proteins are labeled, the ratio of fluorophore to protein, and the specific methodologies employed. Getting these elements right not only enhances visibility but also builds a stronger foundation for subsequent analysis and interpretation of the data.
Selecting the Appropriate Fluorophore
Choosing the right fluorophore is crucial in any protein labeling endeavor. An appropriate fluorophore should have specific characteristics: optimal excitation and emission wavelengths that match the experimental setup, stability under the conditions of use, and minimal background noise. Researchers should be aware of the specific applications they are targeting. For instance, for cellular imaging, one might lean toward fluorophores that fluoresce brightly while exhibiting low toxicity to live cells.
A diverse pool of fluorophores is available, each with its own set of advantages and challenges. Given the context, the researcher may prefer using organic dyes for their tuneable properties or quantum dots for their exceptional brightness and stability. Familiarity with these options helps in making an informed choice that can lead to more discernible results.
Minimizing Background Fluorescence
Background fluorescence can be the bane of any labeling experiment. It muddles the data and can lead to misleading interpretations if not controlled meticulously. Techniques for minimizing background fluorescence include sample preparation methods that promote specificity in binding and employing buffer solutions that reduce autofluorescence.
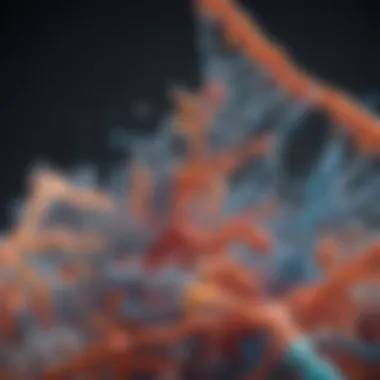
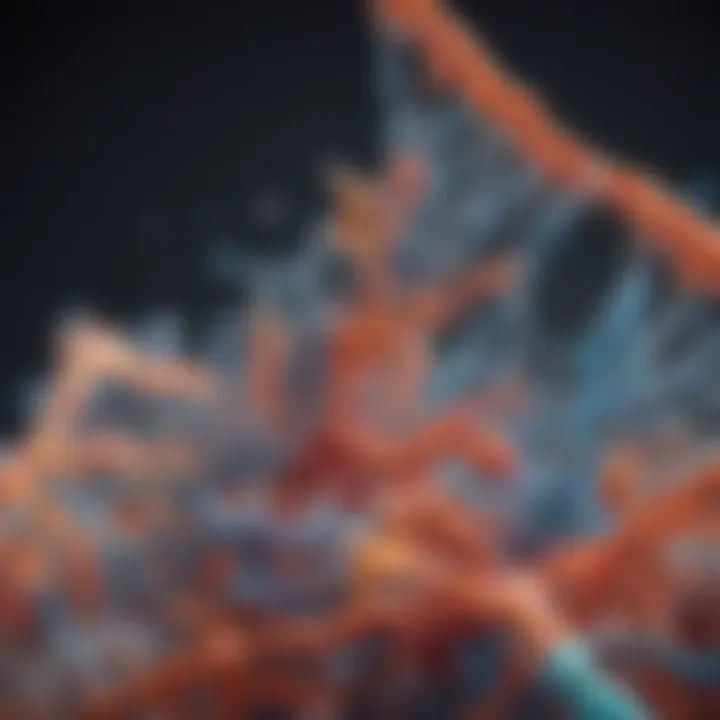
Another useful method is to optimize the washing steps within the protocol to remove unbound fluorophores. These often contribute to unwanted signals that only serve to obscure authentic results. That said, pH levels should also be monitored, as they can influence both the sample’s stability and the fluorophore’s behavior.
Enhancing Signal-to-Noise Ratios
Boosting the signal-to-noise ratio is paramount for high-quality images or data. Achieving this might involve careful calibration of the imaging equipment, as well as adjusting parameters including exposure times and gain settings. Prioritizing well-characterized fluorophores, known for their brightness and photostability, aids in addressing this challenge.
Furthermore, advanced imaging techniques can help. Techniques such as Total Internal Reflection Fluorescence (TIRF) can provide a cleaner signal from the region of interest by minimizing out-of-focus light, thus enhancing the overall quality of the measured fluorescence. In short, optimizing the signal-to-noise ratio can significantly affect the utility of the end results in any biological assays or analyses.
"Effective optimization of labeling protocols not only refines the quality of results but also aids in deriving meaningful conclusions from the data gathered."
In summary, the optimization of labeling protocols is a multi-faceted process that is crucial in ensuring the success of fluorophore labeling in protein research. By focusing on appropriate fluorophore selection, minimizing background fluorescence, and enhancing signal-to-noise ratios, researchers can substantially improve the clarity and reliability of their findings. The pathway to successful protein labeling is meticulous yet rewarding, allowing for a deeper understanding of biological phenomena.
Interpreting Fluorescence Data
Understanding how to interpret fluorescence data is paramount in the realm of protein research. It's not just about getting flashy images under a microscope; rather, it’s a detailed journey into the dynamics of protein behavior within biological systems. Effectively interpreting this data can yield insights into protein interactions, localization, and functionality, transforming the way scientists approach complex biological questions.
Quantitative Analysis Techniques
The cornerstone of interpreting fluorescence data lies in quantitative analysis techniques. This goes beyond mere observation, demanding a rigorous methodology that incorporates precision and consistency. Techniques such as fluorescence intensity measurements, lifetime imaging, and Förster resonance energy transfer (FRET) are just a few methods that provide substantial information about protein dynamics.
- Fluorescence Intensity Measurements: Tracking changes in fluorescence intensity can reveal the concentration of labeled proteins over time. However, one must be cautious, as these measurements can be influenced by various factors, such as photobleaching or overlapping emission spectra.
- Fluorescence Lifetime Imaging Microscopy (FLIM): By measuring the decay time of fluorescence, FLIM allows researchers to distinguish between different environments and interactions. Variation in lifetime can be interpreted to deduce molecular interactions or local environments, providing a deeper level of insight compared to static intensity measurements.
- FRET: This technique offers a unique window into molecular interactions by measuring the energy transfer between two fluorophores. A significant decrease in donor fluorescence intensity when in proximity to an acceptor indicates a successful interaction, making FRET a powerful tool for studying protein-protein interactions.
These techniques necessitate precise calibration and a thorough understanding of the biological context to avoid misleading interpretations. Missteps in this phase can lead researchers down the wrong path, which could prove costly in both time and resources.
Visualization Tools and Software
In today's data-rich environment, the role of visualization tools and software cannot be underestimated. These tools effectively transform complex datasets into comprehensible visual formats that facilitate interpretation.
Some popular software include:
- ImageJ/Fiji: An open-source platform often used for processing and analyzing scientific images. It offers a range of plugins for fluorescence quantification and analysis.
- NIS-Elements: A software package designed for robust image analysis, especially useful in capturing the intricate details of fluorescence signaling.
- GraphPad Prism: A versatile tool ideal for statistical analysis and creating graphs. It allows researchers to see the patterns in their quantitative data clearly.
Using these tools helps bring clarity to data that may initially seem overwhelming. By visually representing the findings, the relationships between different proteins or cellular processes become easier to understand.
"An image can be worth a thousand words, but the right analysis can reveal truths untold."
Selecting appropriate visualization tools depends greatly on the research goals and the complexity of the data at hand. It's crucial for scientists to familiarize themselves with various software options and choose the ones that best fit their specific needs.
Applications of Fluorophore Labeling
The use of fluorophore labeling in protein research has made a remarkable impact on both the scientific community and various fields within biotechnology. It gives a clear lens through which to understand the subtleties of protein dynamics, interactions, and localization within cells. The applications extend across diverse science domains, such as cell biology, biochemistry, and medical diagnostics, showcasing its multifaceted benefits. These insights, coupled with the ongoing innovations in imaging technologies, solidify fluorophore labeling as not just useful but essential in advancing biological research.
Cell Biological Studies
Tracking Protein Localization
Tracking protein localization is pivotal when it comes to understanding cellular functions and the roles proteins play within specific organelles. This method allows researchers to visualize where proteins are situated in living cells, facilitating insights into protein trafficking and organelle dynamics. It’s a reliable strategy, as its precision helps define how proteins interact with their environments in real-time. One of the key characteristics of this approach is its ability to provide spatial context. Knowing where a protein is located can reveal potential interactions and functional states that might not be visible otherwise. Furthermore, this technique often utilizes fluorescent proteins, which integrate seamlessly into biological systems—thus presenting itself as a favored choice for many researchers.
However, a unique challenge accompanies this method. The potential for false interpretations due to variability in localization signals stands out as a concern. The advantages of using specific fluorophores capable of finely tuned signals often offset this issue, but careful consideration is needed during experimental design.
Monitoring Protein Interactions
Monitoring protein interactions expands the understanding of molecular mechanisms within biological systems. This aspect focuses on figuring out how proteins talk to each other, which is critical in signaling pathways and various regulatory mechanisms. By employing techniques such as FRET (Fluorescence Resonance Energy Transfer) or BRET (Bioluminescence Resonance Energy Transfer), it is possible to glean real-time insights into how proteins move and interact in living cells. This powerful tool is becoming increasingly acknowledged for its effectiveness in linking molecular actions to cellular functions.
A notable characteristic of this technique is its capacity to provide qualitative and quantitative data simultaneously. Therefore, it becomes not only a beneficial approach for detailed studies but a popular one as well, yielding significant results in research. Yet, it does come with its drawbacks; for one, it often requires multiple labeling strategies that can complicate the experimental setup and interpretation of results. Choosing the right fluorophores that minimize background fluorescence while ensuring maximum interaction sensitivity is essential for overcoming these hurdles.
Biochemical Analysis
Enzyme Activity Measurements
In biochemical contexts, enzyme activity measurements facilitated by fluorophore labeling play an essential role in understanding catalytic efficiency and kinetics. By coupling enzymatic reactions with fluorescent signals, researchers can track the progress of these reactions in real-time, which opens a window into previously inaccessible dynamics of enzyme action. This effective strategy provides direct insights into how environmental factors or inhibitors affect enzyme activity, proving highly valuable in many experimental setups.
A prominent benefit of using fluorometric assays is their sensitivity. The low detection limits permit even minute changes in enzyme activity to be quantified. However, an area of caution must be noted; the fluorophores can sometimes interfere with enzyme function, possibly skewing results. Despite potential disadvantages, the technique remains a cornerstone in enzyme studies, offering a wealth of information when conducted with care.
Receptor Binding Studies
Receptor binding studies gain from fluorophore labeling by providing clarity on how ligands interact with receptors in a cellular context. This approach is crucial for drug development and understanding signaling pathways, as it allows scientists to witness binding events as they unfold. Such methods frequently employ competition assays using fluorescently labeled ligands to determine binding affinities and kinetics with impressive accuracy.
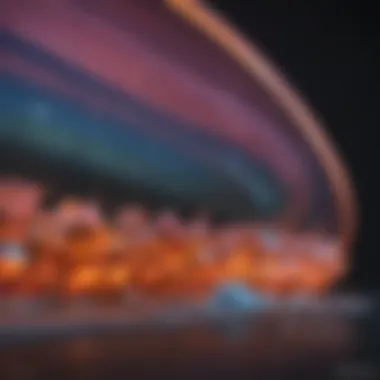
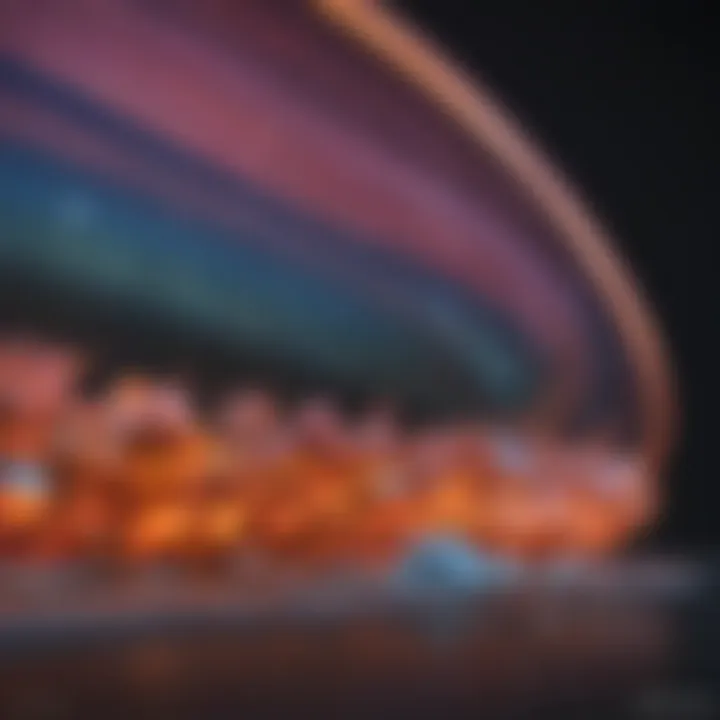
The primary characteristic that makes this technique beneficial is its versatility in different conditions, providing reproducibility across various systems. These advantages contribute to heightened interest and prevalence in pharmacological research. Yet, one should be cognizant of the pitfalls; receptor downregulation or desensitization can alter ligand affinity over time, complicating the interpretation of results. Hence, the implications from receptor binding studies require vigilant analysis and repeated validation.
Diagnostics and Therapeutics
Fluorescence Microscopy in Clinical Settings
In the realm of clinical settings, fluorescence microscopy has transformed how we visualize and diagnose diseases at the cellular level. This technique enables pathologists and clinicians to inspect tissues, cells, and biomolecules with unmatched precision and clarity, leading to enhanced diagnostic capabilities. Fluorophore labeling in clinical diagnostics allows detection of biomarkers linked with various diseases, offering insights into their pathophysiological states.
A defining characteristic of fluorescence microscopy is its ability to differentiate specific cell components with unique fluorescent signatures. This selective labeling enhances diagnostic accuracy, often pivotal in cases of cancer or infections. Nonetheless, the potential for phototoxicity remains a consideration. Care must be taken to mitigate damage to living tissues during prolonged observation, which could otherwise skew clinical assessments.
Drug Development Applications
Fluorophore labeling plays a vital role in drug development eras, particularly in identifying and optimizing lead candidates. It streamlines workflows by allowing researchers to monitor biological responses to therapeutic compounds in real-time. This observation helps discern efficacy, safety, and pharmacokinetic profiles, all crucial for moving forward in the drug discovery process.
A remarkable attribute of this approach lies in its dynamic monitoring, providing responses that static assays might overlook. This quality makes fluorophore labeling a favored strategy among drug developers. However, achieving consistency across diverse experimental conditions can sometimes be a challenge, demanding meticulous protocol optimization to yield reliable results. Successfully navigating these considerations amplifies the potential of drug development applications, reinforcing the importance of this labeling technique in translational research.
Challenges in Fluorophore Labeling
Fluorophore labeling is not without its hurdles. While the technology brings invaluable insights into protein dynamics and interactions, researchers face several challenges that can impact the reliability and quality of their experimental outcomes. Understanding these challenges is crucial for academics and professionals alike, as it allows for more informed decision-making and strategic planning in their experimental designs. Some key elements worthy of consideration include:
- Photobleaching and Phototoxicity
- Labeling Efficiency
- Specificity and Cross-Reactivity Issues
Addressing these challenges not only enhances the credibility of fluorescence-based findings but also contributes to the broader goal of advancing biological research.
Photobleaching and Phototoxicity
Photobleaching occurs when a fluorophore permanently loses its ability to fluoresce due to prolonged exposure to the excitation light. This effect poses a significant challenge in live-cell imaging, where consistent illumination is critical for tracking dynamic processes. When fluorophores are bleached, the data can be misleading, leading to false conclusions about protein interactions or localization.
On the other hand, phototoxicity refers to cellular damage caused by the excitation light itself. This can compromise cell viability and interfere with physiological responses, thereby distorting results. When studying complex cellular behaviors, minimizing both photobleaching and phototoxicity is key. Researchers purposefully select fluorophores that are more stable under light exposure and employ advanced imaging techniques that reduce exposure time or intensity where possible.
"Balancing illumination intensity and exposure time is paramount for preserving specimen integrity while capturing vivid fluorescence."
Labeling Efficiency
The efficiency of fluorophore labeling can vary considerably depending on the method and conditions used. Low labeling efficiency leads to a poor signal, making it difficult to detect the targeted proteins with clarity. This becomes a pressing challenge when conducting quantitative analyses, where accuracy and reproducibility are vital.
Factors affecting labeling efficiency include:
- Fluorophore reactivity and affinity
- Protein folding and conformation
- Reaction conditions
Researchers need to optimize the reaction conditions, such as the concentration of fluorophores and the duration of the labeling reaction, to achieve a higher efficiency. Techniques like site-specific labeling can be employed to increase the precision and consistency of fluorophore attachment, although they may necessitate advanced knowledge of protein biochemistry and molecular biology.
Specificity and Cross-Reactivity Issues
Specificity is crucial when labeling proteins, especially in complex biological systems. Fluorophores can sometimes attach to unintended targets, leading to background fluorescence that obscures the true signal. Cross-reactivity issues arise when the labeling reagents interact with multiple sites or similar proteins within a sample.
This challenge is particularly pronounced in multi-color labeling experiments, where multiple fluorophores are used simultaneously. It is essential to select fluorophores with distinct spectra and to use appropriate controls to verify the specificity of the labeling.
To mitigate these issues, researchers can:
- Perform rigorous validation to ensure specific labeling
- Use advanced imaging techniques to distinguish overlapping signals
- Implement computational methods to deconvolute mixed signals
The Future of Fluorophore Labeling
As we stand at the crossroads of scientific discovery and technological innovation, the future of fluorophore labeling looms larger than life. The continuous evolution in this field holds immense promise, impacting a myriad of areas from basic research to clinical applications. Understanding what lies ahead can fuel progress and inspire innovative solutions to current challenges in protein research.
Innovations in Fluorophore Development
The field of fluorophore development is like an ever-expanding universe, bursting with potential. New fluorophores with increasingly tailored properties are emerging, leading researchers to explore a range of spectral characteristics, brightness, and stability. One significant trend is the shift toward designing synthetic fluorophores that are more resistant to photobleaching, addressing one of the biggest headaches researchers face. These innovations aim to create brighter and longer-lasting labels, giving researchers more time to capture high-quality images of dynamic cellular processes.
Another exciting area is the development of fluorophores that can switch between on and off states—a property known as fluorescence switching. This could potentially allow for real-time monitoring of protein interactions in living systems, offering a fine-grained view of biological processes that occur just under the surface. As researchers refine these switching mechanisms, we’re likely to witness a new wave of fluorescence techniques that can probe deeper into the molecular activities of cells.
The addition of stimuli-responsive fluorophores adds another layer of complexity and versatility. These labels can change their fluorescence properties in response to external stimuli such as light, pH, or ion concentration, offering unprecedented control over protein tracking. Not to mention, the integration of nanomaterials such as graphene and various hybrid systems provide unique surfaces that can enhance fluorescence as well.
Integrating with Advanced Imaging Techniques
Advanced imaging techniques are shaping the future of fluorophore labeling in ways we have yet to fully realize. For instance, combining fluorophore labeling with techniques like super-resolution microscopy pushes the boundaries of what we know about protein localization. This union allows scientists to visualize structures and dynamics at a nanoscale, ultimately unlocking new avenues for discovery.
Moreover, leveraging machine learning with imaging data is becoming increasingly common. Algorithms can now analyze large datasets and identify patterns that might go unnoticed by human eyes. This capability amplifies the effectiveness of fluorophore labeling, enabling previously unimaginable analyses of complex biological interactions over time.
"The integration of smart imaging systems with fluorophore technology can be likened to having a map in uncharted territory; it empowers researchers to navigate through complexities with precision."
Incorporating these advanced techniques with traditional labeling methods also opens up hybrid approaches. This includes the use of fluorophores alongside electron microscopy, enabling correlative microscopy studies that combine the advantages of both worlds. As we advance, we can expect these integrations to refine the granularity and depth of protein studies, providing unparalleled insights.
As we move forward, the ways fluorophore labels can be developed and utilized will inevitably change, illuminating our path in protein research and beyond. Bridging the gaps between innovation and application is crucial, and understanding these future directions offers a roadmap for scientists aiming to contribute to the exciting landscape of protein dynamics.