Exploring Synapses: Structure, Function, and Impact
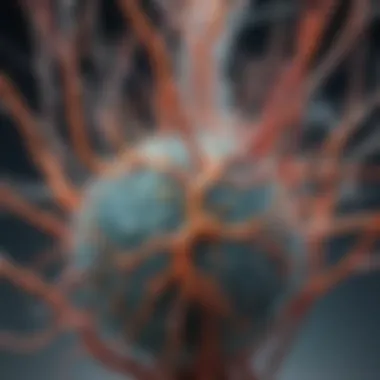
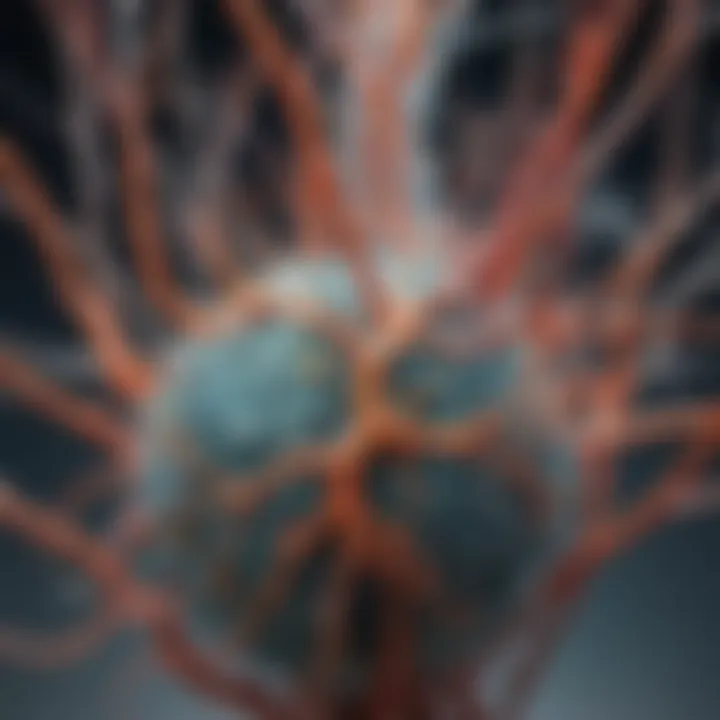
Intro
Synapses, the pivotal junctures in neural communication, bridge the gaps between neurons, facilitating the transmission of information throughout the nervous system. They are more than mere connections; they are dynamic structures that adapt and evolve based on various physiological and pathological conditions. To truly understand the significance of synapses, one must explore their intricate architecture, the intricate dance of neurotransmitters, and the profound implications of their function in both health and disease.
This article aims to paint a comprehensive picture of synapses, delving into their structure, function, and the critical roles they play. By examining the underpinnings of synaptic operations, such as synaptic plasticity and dysfunction, we can gain insights into the larger framework of neural health. Not only will we explore the implications for cognitive processes, but we will also touch on how synaptic impairments contribute to various neurological disorders. Through this exploration, we strive to illuminate the essential nature of synapses and their influence on the broader tapestry of biological processes.
Research Overview
Summary of Key Findings
- Synaptic Structure: Synapses are made up of several key components, including the presynaptic terminal, synaptic cleft, and postsynaptic membrane, each playing a distinct role in neurotransmission.
- Neurotransmission: The release of neurotransmitters from the presynaptic neuron into the synaptic cleft allows communication with the postsynaptic neuron, ultimately influencing neuronal activity.
- Synaptic Plasticity: This characteristic enables synapses to strengthen or weaken over time, depending on activity levels, which is crucial for learning and memory.
- Implications for Neurological Diseases: Disruptions in synaptic function are linked to a range of disorders, highlighting the need for targeted therapeutic interventions.
Background and Context
Understanding synapses is essential for grasping how the brain operates on a fundamental level. These structures do not merely transmit signals; they modulate and refine the very nature of neuronal communication. The study of synapses dates back to the late 19th century, when early neuroscientists proposed the neuron doctrine, establishing that neurons are discrete cells communicating through specialized contact points.
As research progressed, the microscopic examination of synapses unraveled their complex organization. Modern imaging techniques now allow scientists to visualize synaptic activity in real-time, offering profound insights into the mechanisms that govern these interactions.
Methodology
Experimental Design
To investigate synaptic function and structure, researchers often employ a combination of in vivo and in vitro approaches. Animal models, such as rodents, are frequently used to study synaptic dynamics in a living system. These models allow scientists to observe the effects of various interventions and diseases on synapse function.
Data Collection Techniques
Data collection in synaptic research typically includes:
- Electrophysiology: Records electrical activity in neurons to measure synaptic efficacy.
- Imaging Techniques: Techniques like two-photon microscopy permit the visualization of synaptic activity at an unprecedented scale.
- Molecular Biology: Tools such as CRISPR for gene editing allow researchers to manipulate specific genes that are relevant to synaptic function.
Understanding these methodologies is crucial for interpreting findings and their implications in synaptic research.
Prolusion to Synapses
Synapses are often considered the cornerstone of neural communication. This section aims to outline the significance of understanding synapses, as they not only facilitate the exchange of information between neurons but also play a crucial role in shaping the brain’s workings. Whether we’re learning, remembering, or processing our surroundings, synapses are at the heart of these processes. Knowing how they function can open doors to numerous insights, especially in the realms of neuroscience and psychology.
By diving into the details of synaptic structure and function, readers can grasp how tiny gaps are anything but trivial; they represent sites where both electrical and chemical signals mingle, significantly influencing how our nervous system operates. Studying synapses is like peering through a keyhole into the vast world of neural networks, providing understanding into everything from motor control to emotional regulation.
Definition and Overview
A synapse is essentially the junction where one neuron communicates with another. There are different types of synapses, primarily classified as chemical and electrical. Chemical synapses are the more common of the two, involving neurotransmitter release into the synaptic cleft, which then binds to receptors on the postsynaptic neuron. In contrast, electrical synapses allow direct transmission of electrical signals through gap junctions, providing almost instantaneous communication but with less versatility.
The basic components of a chemical synapse include:
- Presynaptic terminal: This is where neurotransmitters are stored and released. It's packed with vesicles filled with chemical messengers.
- Synaptic cleft: A minute gap between the presynaptic and postsynaptic neurons, typically around 20-40 nanometers wide.
- Postsynaptic membrane: This contains receptors which recognize and bind the neurotransmitters sent from the presynaptic neuron.
In summary, understanding synapses involves unraveling the interplay of these components, uncovering how they contribute not just to communication, but also to learning, memory, and overall brain function.
Historical Perspectives
The exploration of synapses has a rich history that traces back to the early days of neuroscience. Early thinkers like Santiago Ramón y Cajal, often crowned as the father of modern neuroscience, laid the groundwork by utilizing staining techniques to visualize neurons and their intricate relationships. He proposed the neuron doctrine, which positioned these cells as the fundamental units of the nervous system, marking a pivotal shift in how we see brain organization.
As science progressed, the concept of the synapse evolved. In the early 20th century, Charles Sherrington introduced the term "synapse," positing that it acts as a functional interface between neurons. His work delineated how synaptic connections can either facilitate or inhibit neural impulses, leading to a nuanced understanding of reflexes.
Fast forward to the late 20th century, and research around synapses flourished, especially with the advent of advanced microscopy techniques. These developments allowed scientists to visualize synaptic activity in real time, revealing complex processes like synaptic plasticity—which is integral to learning and memory. Such milestones have propelled not just neuroscience as a field but have also infiltrated discussions around psychiatric conditions and neurodegenerative diseases.
Consequently, exploring the synapse is an ongoing journey, a narrative deeply rooted in history and one that continues to evolve. From Cajal's drawings to today's cutting-edge techniques, each discovery builds upon the last, enriching our understanding of these vital structures.
The Anatomy of Synapses
Understanding the anatomy of synapses is critical to comprehending how neuronal communication occurs within the brain. Synapses serve as the essential junctions where signals are transmitted from one neuron to another, facilitating the transfer of information. This architecture is not just a patchwork of cells; it reflects the intricate dance between structure and function that underpins neural connectivity and, ultimately, all aspects of behavior and cognition. By examining the anatomy, we reveal the specific roles each component plays in neurotransmission, synaptic plasticity, and the overall functionality of nervous systems.
Presynaptic Structure
Terminal boutons
The terminal boutons, or synaptic terminals, are specialized endings of axons that play a pivotal role in neurotransmission. These structures are often described as the command centers of synaptic communication due to their function in releasing neurotransmitters into the synaptic cleft. One key characteristic of terminal boutons is their rich abundance of synaptic vesicles, which store neurotransmitters until they are ready for release.
What's particularly beneficial about terminal boutons is their ability to convert electrical signals (action potentials) into chemical signals efficiently. When an action potential reaches the bouton, it triggers the opening of voltage-gated calcium channels. Calcium ions flood into the terminal, resulting in the exocytosis of neurotransmitter-filled vesicles. This process is crucial because it allows for the rapid communication between neurons, a necessity in both reflex actions and complex cognitive tasks.
A unique feature of terminal boutons is their dynamic adaptability. They can develop new synaptic connections in response to activity, which is a fundamental aspect of learning and memory. However, one could argue that excessive plasticity might lead to dysregulation in neurotransmitter release, potentially contributing to neuropsychiatric disorders.
Synaptic vesicles
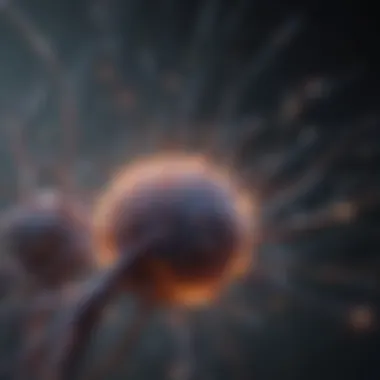
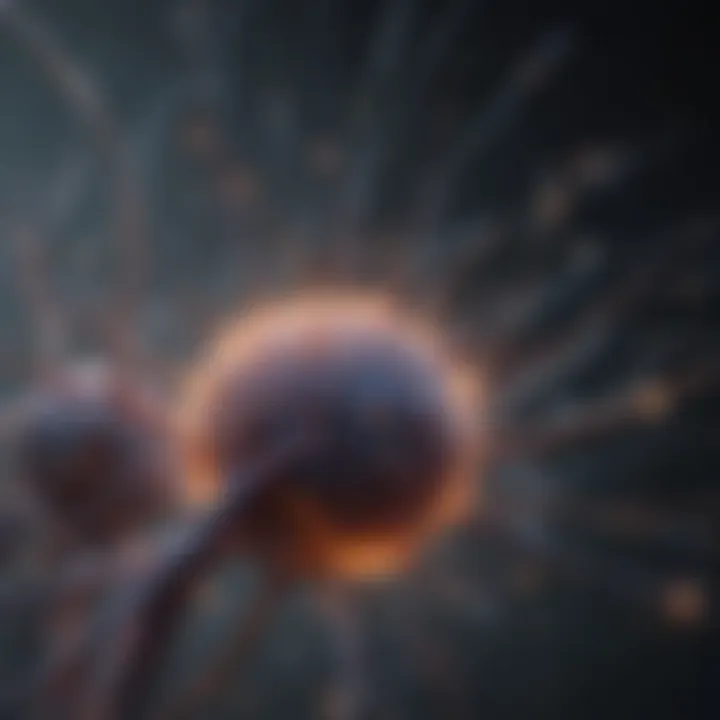
Synaptic vesicles are undeniably essential to synaptic transmission, as they carry neurotransmitters to the presynaptic membrane. These tiny sacs can hold different types of neurotransmitters, each contributing differently to synaptic communication. Their key characteristic lies in their size and the lipid bilayer that encapsulates them, enabling them to merge seamlessly with the presynaptic membrane when calcium levels rise during action potentials.
What makes synaptic vesicles a popolar choice for research is their role in neurotransmitter modulation. The mechanisms behind vesicle loading and release have been extensively studied, shedding light on their role in both synaptic strength and versatility. A unique aspect of these vesicles is the process of replenishment, whereby reserve pools of vesicles can be drawn upon to maintain neurotransmitter release during sustained neuronal activity.
Yet, there are some disadvantages to consider. If there is a malfunction within vesicle cycling or abnormal release rates, it can lead to various neurological and psychiatric conditions. The interplay between synaptic vesicles and the presynaptic structure is precarious yet vital for healthy neural function.
Postsynaptic Structure
Dendritic spines
Dendritic spines are small, protruding structures on dendrites that serve as the primary sites for synaptic inputs. These spines increase the surface area for neurotransmitter receptors, thus enhancing the ability of the postsynaptic neuron to receive signals. What's notable is that dendritic spines can change their shape and size in response to activity, a phenomenon that underscores their importance in synaptic plasticity.
Their adaptability to various synaptic environments makes them a beneficial focus for this article. By examining how spines adjust to different stimulation frequencies or patterns, researchers can gain insights into memory and learning processes. However, if dendritic spines become too numerous or malformed, they can disrupt normal synaptic transmission, leading to cognitive impairments.
Receptors
Receptors are fundamental components of the postsynaptic structure. They bind neurotransmitters released from the presynaptic terminal, translating chemical signals back into electrical signals within the postsynaptic neuron. A key characteristic of receptors is their diversity; ionotropic receptors allow for fast synaptic transmission, while metabotropic receptors engage in slower, but longer-lasting, synaptic modulation.
In this article, focusing on receptors is significant due to their direct involvement in shaping neuronal responses to incoming signals. For instance, the specific binding affinity of a receptor can determine how effectively it responds to a neurotransmitter, impacting everything from reflex responses to emotional regulation.
One unique feature of receptors is their capacity for desensitization; after prolonged exposure to a neurotransmitter, many receptors reduce their responsiveness. While this can protect against over-stimulation, it can also lead to issues in synaptic plasticity, particularly in neuronal networks associated with learning and memory.
The Synaptic Cleft
The synaptic cleft, albeit a tiny space, is a crucial and dynamic region that separates the presynaptic and postsynaptic neurons. Measuring approximately 20-40 nanometers wide, it serves as the medium through which neurotransmitters diffuse after being released from synaptic vesicles. Its importance lies in its role as a battlefield for neurotransmitter transport and receptor engagement.
In this narrow gap, neurotransmitter concentrations can fluctuate sharply, influencing the strength and duration of synaptic signals. The cleft is not passive; rather, it can impose physical and chemical constraints that affect how effectively substances travel from one side to the other. For example, the size and structure of the synaptic cleft can change during certain physiological conditions, such as learning—creating a prime example of structural plasticity.
The dynamics occurring within the synaptic cleft are complex. Factors like enzymatic degradation of neurotransmitters and nearby astrocyte involvement in neurotransmitter uptake are pivotal in terminating the signal between neurons. Having a thorough understanding of the synaptic cleft is essential for grasping the entire scope of synaptic communication, and it serves as an important area for investigating synaptic dysfunction in various neurological disorders.
Mechanisms of Synaptic Transmission
Understanding the mechanisms of synaptic transmission is key to grasping how neurons communicate and develop networks throughout the nervous system. This intricate process involves several steps that are vital for transmitting signals efficiently. Each component plays a specific role, from the release of neurotransmitters to receptor activation and finally, signal termination. Learning about these mechanisms not only highlights their complexity but also underscores their importance in broader biological functions and potential therapeutic applications.
Neurotransmitter Release
Calcium influx
Calcium ions serve as the steady hands of a conductor orchestrating the symphony of neurotransmitter release. When an action potential reaches the presynaptic terminal, it triggers an influx of calcium ions into the nerve terminal through voltage-gated calcium channels. This surge in calcium is pivotal as it initiates processes necessary for the release of neurotransmitters. One key characteristic of calcium influx is its rapidity. It allows the reaction to happen almost instantaneously, reflecting the urgency of neuronal communication. This quality makes it a favorite topic within the realm of synaptic transmission studies because it illustrates how cells can relay information at lightning speed.
Moreover, calcium ions have a unique feature in this process—their concentration gradient. When calcium enters the neuron, it encourages synaptic vesicles to fuse with the presynaptic membrane and release their neurotransmitter contents into the synaptic cleft. However, there can be disadvantages to relying heavily on calcium. For instance, over-excitation due to excessive calcium can lead to cellular damage and contribute to various neurological disorders.
Exocytosis
Exocytosis is the curtain call of neurotransmitter release, where the synaptic vesicles take center stage to discharge their cargo into the synaptic cleft. As vesicles fuse with the presynaptic membrane, they release neurotransmitters that float across the synaptic gap to activate their respective receptors. One of the standout characteristics of exocytosis is that it’s not merely a one-and-done affair; rather, it's a finely tuned process influenced by factors such as calcium levels and cellular environment. This makes exocytosis a popular topic within synaptic studies, for it provides a window into how neurons communicate and adapt to varying conditions.
The unique aspect of exocytosis lies in its granularity. Different neurotransmitters can be released in varying amounts, showcasing how adaptable this system is in meeting the demands of neural communication. Yet, not everything is rosy. Misregulated exocytosis can lead not only to signal transmission deficiencies but also to phenomena such as synaptic fatigue.
Receptor Activation
Ionotropic receptors
Ionotropic receptors are akin to the front doors of a busy nightclub, swinging open under the right conditions to allow guests—or ions, in this case—into the party. These receptors transform the chemical signals from neurotransmitters into electrical currents almost instantly. A significant feature of ionotropic receptors is the speed of their action—they produce quick, transient effects. This attribute makes them an essential player in fast synaptic transmission, which is crucial for processes such as reflexes and sensory perception.
The unique feature that makes ionotropic receptors particularly interesting is their structural simplicity. They form a channel that opens when a neurotransmitter binds, allowing specific ions like sodium or potassium to pass through. However, their rapid response comes with a trade-off. They can sometimes lead to desensitization, where, after repeated stimulation, the receptors become less responsive to their neurotransmitter.
Metabotropic receptors
Metabotropic receptors are the cerebral thinkers of synaptic transmission. Unlike their faster counterparts, they operate more slowly but have far-reaching effects that can modify the behavior of the neuron over longer periods. One distinguishing characteristic of metabotropic receptors is their reliance on second messengers. When activated by neurotransmitters, they initiate a cascade of intracellular signaling events that can change various neuronal functions over time.
This ability to influence a wide array of biological activities lends metabotropic receptors special significance. They are particularly discussed in the context of long-term changes and synaptic plasticity, because they allow the system to adapt and refine itself based on past activity. However, the complexity of their signaling pathways can often be a double-edged sword, as dysregulation can lead to complex disorders such as mood and anxiety issues.
Signal Termination
Reuptake mechanisms
Reuptake mechanisms function like the cleanup crew after a big event, ensuring that leftover neurotransmitters are efficiently removed from the synaptic cleft. This process involves the reabsorption of neurotransmitters back into the presynaptic neuron, thus terminating the signal. The key characteristic of reuptake is its high specificity—transporters are often selective for particular neurotransmitters, allowing for targeted adjustments in neuronal signaling. This makes reuptake mechanisms a potent tool in controlling synaptic strength.
The unique aspect here is the modulation of neurotransmitter availability. By controlling how long neurotransmitters remain in the synaptic cleft, reuptake mechanisms influence not only immediate signaling but also longer-term changes. Yet, over-reliance on this mechanism can result in uneven neurotransmitter levels, which is implicated in various neurological and psychiatric disorders.
Enzymatic degradation
Enzymatic degradation is the biochemical equivalent of a gatekeeper, ensuring that neurotransmitters do not linger unnecessarily in the synaptic cleft. Different enzymes break down neurotransmitters into inactive components, allowing for the clearing of signals when communication is no longer needed. A distinct characteristic of enzymatic degradation is its efficiency—specific enzymes can rapidly metabolize neurotransmitters, effectively resetting the synapse for the next wave of action.
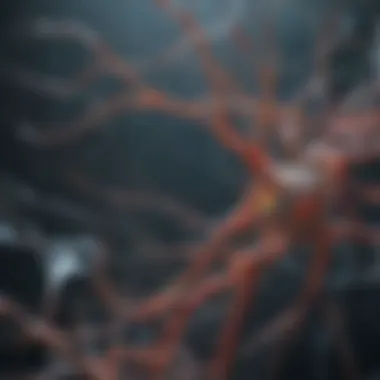
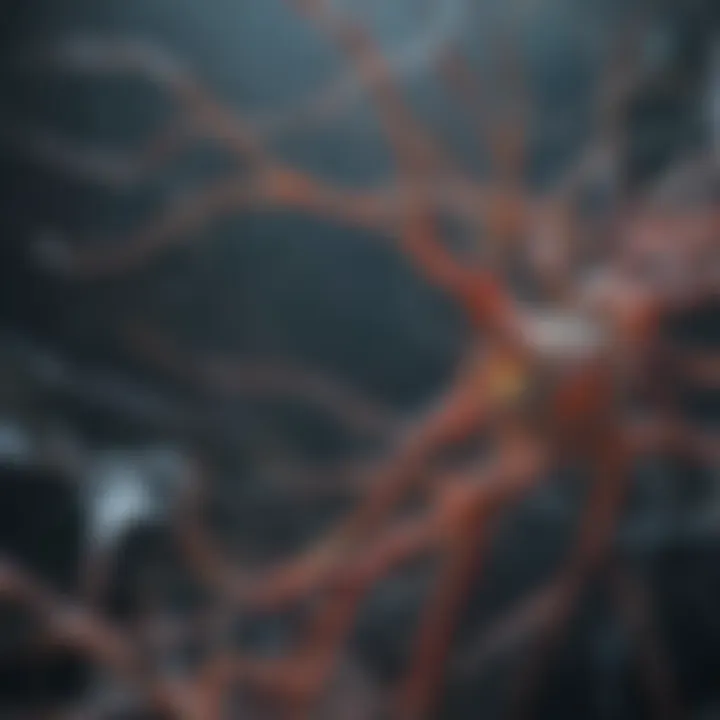
The most renowned example is acetylcholinesterase, which swiftly breaks down acetylcholine in the synaptic cleft. This process can be beneficial, as it provides precise control over synaptic transmission. However, when enzymes malfunction, it can lead to severe consequences, such as persistent signals contributing to muscle spasms and other disorders.
Understanding these mechanisms not only uncovers the hidden intricacies of neural communication but also provides crucial insight into potential targets for therapeutic interventions.
Synaptic Plasticity
Synaptic plasticity is a cornerstone concept in understanding how the brain adapts to experiences and learning. This biological phenomenon refers to the ability of synapses, the junctions between neurons, to strengthen or weaken over time in response to increases or decreases in their activity. Plasticity is vital for various processes such as memory formation and adaptation to new information; it is like the brain's way of fine-tuning the inputs and outputs among its vast neuronal connections.
The implications of synaptic plasticity extend far beyond mere memory; they touch upon our ability to adapt to changes, recover from injuries, and potentially even reverse some neurological disorders. Hence, a comprehensive exploration of this topic not only informs us about fundamental neural mechanisms, but it highlights synaptic plasticity's critical involvement in health and disease.
Types of Plasticity
Long-term potentiation
Long-term potentiation (LTP) is a prime player in the realm of synaptic plasticity. It describes the persistent strengthening of synapses based on recent patterns of activity. As neurons communicate, certain pathways become more effective at transmitting signals. The key characteristic of LTP lies in its longevity; it doesn’t just happen at a whim, but instead reflects a synapse’s history of use. Through LTP, synapses take on a kind of memory, which is instrumental in learning and retaining information.
One unique aspect of LTP is its reliance on calcium ions. When the presynaptic neuron fires, calcium ions rush into the postsynaptic neuron, triggering a cascade of signaling events. This is a functional potent tool for researchers and educators, as understanding LTP can pave the way for cognitive enhancement strategies. Yet, it’s noteworthy to consider the potential downsides: excessive LTP can also be responsible for pathological conditions, such as chronic pain or certain neurodegenerative disorders, showing that while beneficial, it must be kept in check.
Long-term depression
Conversely, we have long-term depression (LTD), which involves the long-lasting decrease in synaptic strength following low-frequency stimulation of a synapse. LTD is equally as crucial as LTP in regulating synaptic efficacy, and its role is often painted as a balancing act to the potentiation process. The key characteristic of LTD is its ability to aid in forgetting irrelevant information, allowing a renewed focus on newly relevant data.
The unique feature of LTD is its effects on receptor density. In response to stimuli that do not meet a certain threshold, the postsynaptic neuron may reduce the number of available receptors or the effectiveness of synaptic transmission. This is an insightful point for the article; understanding the nuances of LTD rounds out our knowledge of synaptic plasticity's role in learning and memory. However, like LTP, there are drawbacks. LTD can contribute to cognitive decline in the context of disorders like Alzheimer’s.
Molecular Mechanisms
Role of calcium
Calcium ions play a significant role in various synaptic processes, acting as a second messenger in extensive signaling pathways. The influx of calcium into neurons can trigger numerous biochemical cascades, leading to changes in synaptic strength. This facet underlines the importance of calcium in both LTP and LTD processes, making it a linchpin in synaptic functioning.
The benefit of focusing on calcium’s role is clear: it underscores the crucial intersection of electrical and biochemical signaling in the nervous system. This understanding has potential therapeutic implications as targeting calcium channels or pathways may offer novel avenues for treatment in diverse neurological disorders. Recent studies hint at how manipulating calcium dynamics could be essential for synaptic health, but this complexity can also introduce challenges in drug design and efficacy.
Synaptic proteins
Another key player in synaptic plasticity is a range of proteins that facilitate and regulate synaptic functions. Synaptic proteins, such as glutamate receptors, scaffolding proteins, and signaling molecules, contribute to synaptic formation and remodeling - they are the workhorses behind the scenes. Highlighting these proteins adds depth to the discussion on synaptic plasticity since they dictate how synapses respond to changes in activity.
The importance of synaptic proteins can't be overstated. When examining synaptic alterations due to experience, these proteins shine a light on the biological mechanisms that underlie learning and memory. Moreover, studying them can reveal how dysregulation leads to profound neurological impairments. However, one must also note that the overexpression or mutation of certain synaptic proteins might correlate with various pathologies, making it crucial to zero in on their functions and interactions within this dynamic network.
Impact of Synaptic Dysfunction
The ramifications of synaptic dysfunction in the brain extend far beyond the mere failure of neurotransmission; they often signify the onset of various neurological and psychiatric disorders. Understanding the impact of these disruptions is essential, not only for grasping the mechanics of such diseases but also for devising potential therapeutic strategies. Synapses serve as the critical communication hubs among neurons, orchestrating the flow of information necessary for every thought, movement, and emotion. When these intricate networks falter, it can create a cascade of effects that permeate numerous aspects of an individual's health.
Neurological Disorders
Alzheimer’s Disease
Alzheimer’s disease represents a compelling case study when examining synaptic dysfunction. This neurodegenerative condition is primarily characterized by the progressive loss of synapses, which leads to cognitive decline. The decline of synaptic connections is linked closely to memory loss and impairment in other cognitive functions. What makes Alzheimer’s particularly noteworthy is its early onset of synaptic failure, setting the stage for a larger decline in neural integrity. The unique hallmark of Alzheimer's is the formation of amyloid plaques and tau tangles, disrupting synaptic function as these proteins accumulate. This distinctive feature allows for targeted research into potential interventions aiming to halt or reverse the synaptic degradation, making it a focal point in the discussions surrounding synaptic health and disease.
Parkinson’s Disease
Parkinson’s disease also provides rich insight into the impacts of synaptic dysfunction. Primarily marked by motor dysfunction, the disease is a result of dopaminergic neuron loss, which profoundly affects synaptic signaling in motor pathways. A key characteristic of Parkinson’s is the presence of Lewy bodies, which disrupt synaptic integrity within the basal ganglia, imposing challenges on voluntary movement. This specific aspect of Parkinson’s gives it emblematic status among synaptic diseases, emphasizing the importance of neurotransmitter balance. Its unique intertwining of motor and cognitive symptoms presents a compelling perspective on how synaptic dysfunction can influence broad ranges of neural networks, necessitating comprehensive therapeutic approaches that address both motor and non-motor symptoms.
Psychiatric Conditions
Schizophrenia
When considering psychiatric conditions, schizophrenia stands out for its complex relationship with synaptic dysfunction. This disorder exhibits symptoms such as delusions and cognitive impairments rooted in disrupted dopamine signaling. A notable characteristic of schizophrenia is the hypofunction of glutamatergic transmission, suggesting a critical role in mood regulation and perception. This facet makes schizophrenia an intriguing focus in understanding how synapses may contribute to broader neural circuitry issues. The distinct combination of cognitive and perceptual anomalies provides a unique opportunity to explore how interventions aimed at synaptic restoration may aid in alleviating symptoms, enhancing the overall quality of life for affected individuals.
Depression
Depression, too, is significantly influenced by synaptic health, where serotonergic and noradrenergic dysfunction plays pivotal roles. Major depressive disorder is often characterized by decreased synaptic plasticity, making it difficult for individuals to forge new neural connections essential for mood regulation. Highlighting this condition’s unique feature of neuroplasticity challenges, it provides insights into therapeutic measures like antidepressants that aim to enhance synaptic function and promote neurogenesis. The understanding of depression through the lens of synaptic dysfunction sheds light on the potential for more tailored interventions, bringing forth innovative research to tackle this pervasive issue in mental health.
Research Methodologies in Synaptic Studies
Understanding synapse structure and function hinges on rigorous research methodologies. These approaches are essential to unraveling the complexities of synaptic communication and observing how these microscopic structures participate in broader brain functionality. Various techniques have emerged, each offering unique insights into synaptic behaviors and properties. The methodologies discussed here are delightfully sophisticated and offer significant contributions to the study of neurotransmission.
Imaging Techniques
Electron microscopy
Electron microscopy has become a cornerstone in the field of synaptic studies. It allows scientists to visualize synapses at a resolution that is simply unattainable with light microscopy. This technique uses beams of electrons instead of light to dissect cellular structures, yielding detailed images of synaptic anatomy. This clarity helps researchers observe the intricate formations of synaptic boutons and the arrangement of neurotransmitter vesicles.
One of the standout features of electron microscopy is its unrivaled resolving power, which reveals synaptic microstructures. Because it allows for such detailed observations, it’s a popular choice in studies aimed at understanding synaptic morphology and alterations in various pathological states. However, it does come with its drawbacks, such as the necessity for complex sample preparation and potential damage to the specimen. Despite these obstacles, the wealth of information it provides is worth the trade-offs.
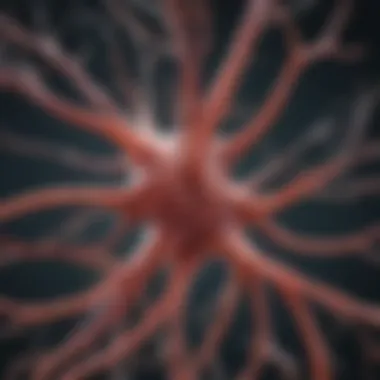
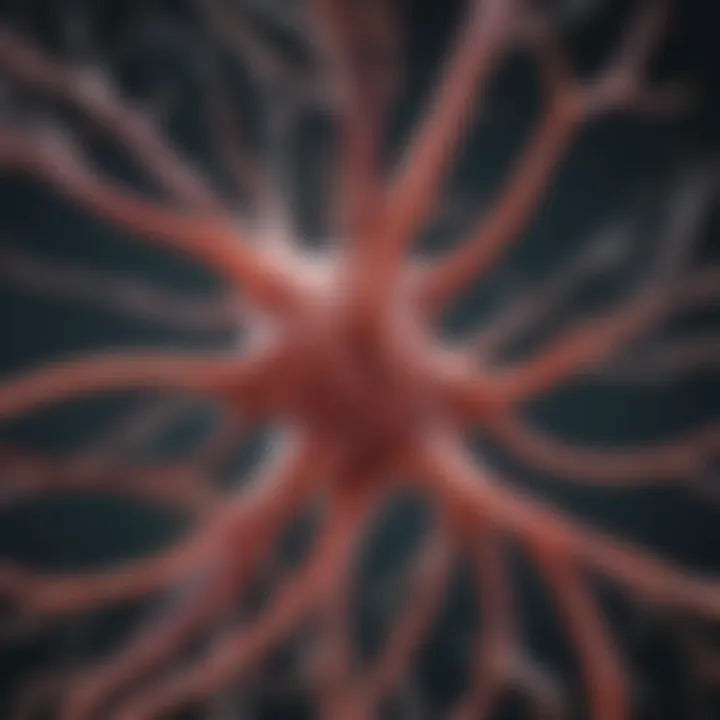
Fluorescence microscopy
On the flip side, fluorescence microscopy offers dynamic insights into synaptic function over time. This technique uses fluorescent markers to tag specific proteins or other molecules within the synapse, illuminating them under specific wavelengths of light. It’s particularly useful when studying the real-time interactions of neurotransmitters and their receptors, giving a semi-live context to the often static visualizations of electron microscopy.
One of the key characteristics of fluorescence microscopy is its ability to image living cells, providing insights into dynamic synaptic processes in their native environment. Its unique feature is the ability to utilize multiple fluorophores simultaneously, allowing researchers to observe various components within a synapse concurrently. However, while it does offer substantial benefits, accurate quantification can be a bit trickier due to overlapping signals from fluorescent tags.
Electrophysiological Techniques
Patch-clamp technique
Patch-clamp technique gives a front-row seat to synaptic activity by measuring ion currents flowing through individual ion channels in the membrane of neurons. This powerful method enables precise control over the intracellular environment while monitoring how synaptic transmission plays out at a very granular level. The standout quality of patch-clamping is its ability to isolate specific currents, providing clarity about the timing and type of synaptic events as they unfold.
Because of its precision, this method is considered a pillar in studies of synaptic transmission. Yet, one must also grapple with its limitations. It often requires specialized training and can be limited by cell viability—the process of obtaining a good seal on the cell membrane can potentially compromise the cell's health, affecting the results obtained.
Field recordings
Field recordings, in contrast, allow for the assembly of information from populations of cells, painting a broader picture of synaptic transmission across networks. This technique records electrical activity in a defined area of tissue, delivering insights into synchronous activity and overall network dynamics. It is particularly useful for studying how synaptic networks function together during particular tasks or in response to stimuli.
Field recordings’ strength lies in their ability to assess the collective behavior of multiple neurons. However, the generalized data can sometimes mask individual cellular responses or pathways, making it a balancing act between macro-level analysis and micro-level resolution.
Research methodologies are pivotal for advancing our understanding of synaptic behavior. They help bridge the gap between the microscopic and functional aspects of synapses, feeding into a broader narrative about brain functionality.
Therapeutic Implications
Understanding the therapeutic implications of synaptic research is critical for the advancement of neurological and psychiatric treatments. Synapses are not merely conduits for signals; they are dynamic entities that can be manipulated to foster recovery from diseases or enhance cognitive function. This section will delve into various methodologies designed to target synaptic transmission, providing a clearer perspective on how such approaches can alleviate synaptic dysfunction and improve patient outcomes.
Targeting Synaptic Transmission
Drugs for neurotransmitter modulation
Drugs that modulate neurotransmitter activity are at the forefront of therapeutic interventions. These medications aim to influence the levels and action of neurotransmitters, enhancing or inhibiting synaptic transmission to alleviate symptoms of disorders like depression or schizophrenia. A significant characteristic of these drugs is their targeted approach; they can selectively enhance or block specific neurotransmitter systems, which can lead to tailored treatment regimens for patients.
One widely known example is selective serotonin reuptake inhibitors (SSRIs), which increase serotonin levels in the synaptic cleft. This regulation of neurotransmitter dynamics is a popular choice due to its proven effectiveness in many patients. However, the unique feature of these drugs lies in their ability to lead to side effects, which can impact patient compliance and long-term success. Additionally, the timing and dosage are crucial in determining their efficacy versus potential adverse effects.
In summary, while drugs for neurotransmitter modulation present a beneficial route for treatment, they require careful consideration and tailored application to maximize their effectiveness without compromising patient well-being.
Gene therapy approaches
Gene therapy stands out as a promising, cutting-edge avenue in targeting synaptic dysfunction. Rather than just modulating existing neurotransmitter levels, this approach aims to address the root cause by altering the genetic material in neurons to promote proper synaptic function. This innovative strategy can lead to more durable outcomes, especially for disorders with a strong genetic component.
The key characteristic of gene therapy is its long-lasting effects—aiming to produce a more significant impact than traditional pharmaceuticals. For example, a recent study highlighted the potential of gene delivery systems to correct deficits experienced in conditions like Alzheimer’s disease by enhancing synaptic function and plasticity. However, the unique feature of gene therapy approach is also its complexity and the ethical concerns surrounding genetic modifications, which are substantial barriers that need consideration.
Thus, while gene therapy approaches provide a fascinating potential for developing new treatments, these come hand-in-hand with challenges that must be navigated cautiously to ensure safe and effective applications.
Future Directions in Research
As we look ahead, the study of synapses paves the way for a variety of exciting avenues in research and application. Understanding how synapses develop and function over time could unveil new perspectives on treatment strategies that could radically change patient care in neurology and psychiatry.
Understanding synaptic development
Understanding synaptic development remains a vital point of interest in the field. It encompasses how synapses form, mature, and adapt based on experiences throughout an individual’s life. This aspect of research can illuminate not only the underlying mechanistic principles of learning and memory but also help tease apart issues related to developmental disorders like autism.
A prime advantage of investigating synaptic development is its potential to lead to early interventions. By identifying critical periods of synaptic growth and plasticity, researchers could potentially harness therapeutic strategies to promote healthier neural connections. However, the downside is that manipulation during these sensitive stages must be approached with great caution, as it could have unintended effects on overall neurodevelopment.
Modeling synaptic networks
Modeling synaptic networks is another promising area of future research. This involves creating computational and biological models to simulate how different synapses interact within neural circuits. Such models can provide crucial insights into the collective behavior of neurons, helping researchers predict how synaptic changes can influence overall brain activity.
The unique feature of network modeling is its ability to incorporate data from various sources, enabling a more robust understanding of complex systems. This approach can offer explanations for phenomena like memory formation and retrieval or the effects of synaptic disruptions. Nevertheless, achieving an accurate representation includes considerable challenges, especially in terms of scalability and the diversity of neuronal properties.
Closure
The exploration of synapses is not merely an academic exercise but a vital inquiry into the mechanisms that underpin both normal and pathological processes in the nervous system. As the junctions through which neurons communicate, synapses facilitate an array of functions, from the simplest reflex actions to the most complex cognitive tasks. Consequently, understanding their structure and function can illuminate how our brains operate at a fundamental level.
Summary of Key Points
In this article, we delved into several key aspects of synapses:
- Structure of Synapses: We examined the intricate architecture comprising presynaptic and postsynaptic components, including neurotransmitter vesicles and receptor sites. The synaptic cleft's role as the intermediate space in signaling was also outlined.
- Transmission Mechanisms: The processes governing neurotransmitter release, receptor activation, and subsequent signal termination were discussed, showcasing how information travels across synapses.
- Plasticity and Dysfunction: We highlighted synaptic plasticity, emphasizing its critical role in learning and memory, while also addressing the consequences of synaptic dysfunction in conditions such as Alzheimer's and schizophrenia.
- Research Methodologies: Different techniques used to study synapses, including advanced imaging and electrophysiological methods, were analyzed, demonstrating the evolving landscape of synaptic research.
- Therapeutic Implications: We looked at potential medical applications stemming from synaptic research, such as drug development and gene therapy approaches, giving a perspective on the future of treatments targeting synaptic mechanisms.
The Importance of Synaptic Research
Synaptic research stands at the forefront of neuroscience, offering profound implications for understanding mental health and neurological disorders. Every breakthrough in this field enhances our grasp of how synapses contribute not just to isolated functions like motor skills or sensory perception but also to broader cognitive abilities and emotional states.
In a world where brain-related illnesses are on the rise, the significance of synaptic studies becomes even clearer. From research-intensive methodologies to clinically inspired insights, each facet contributes to a holistic understanding of the brain. Further inquiry can lead to the development of specialized therapeutics tailored for those suffering from synaptic dysregulations, potentially transforming lives.
Continued exploration of synaptic dynamics is essential for both fundamental and applied neuroscience, bridging the gap between mechanistic insights and clinical applications.
As we wrap up this comprehensive discussion, it is evident that synapses are not just points of contact between neurons but are pivotal players in the intricate ballet of thoughts, movements, and emotions that define our humanity.