In-Depth Analysis of SSDNA Synthesis and Advances
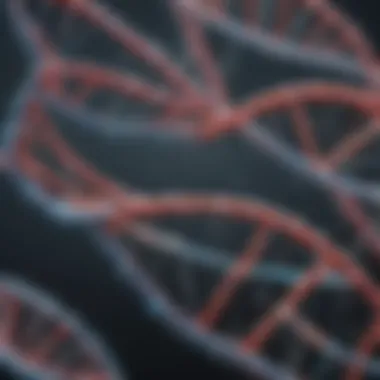
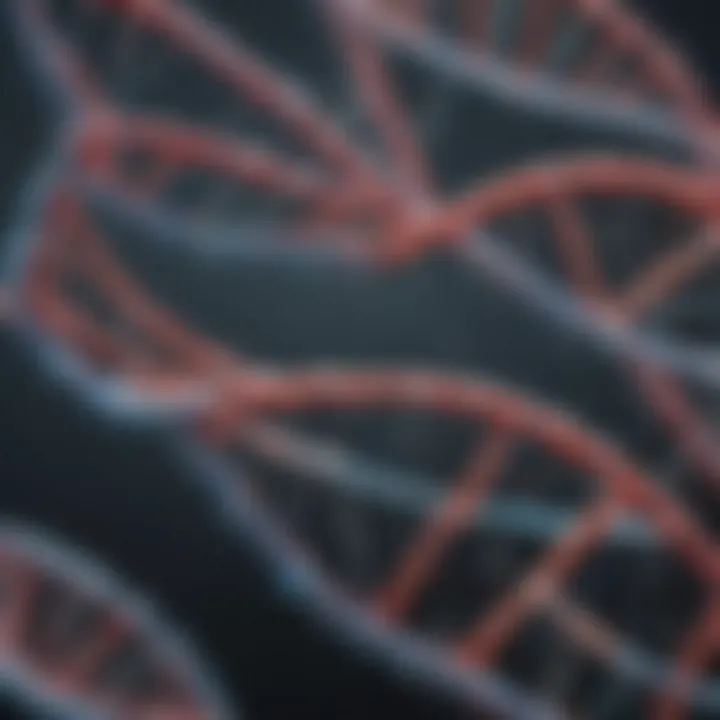
Intro
Single-stranded DNA (ssDNA) plays a significant role in modern biotechnology. Its synthesis is essential in various fields, including genetics, diagnostics, and synthetic biology. Understanding the mechanisms that underlie ssDNA synthesis allows researchers to explore innovative applications and enhance existing technologies. This article delves into the processes involved in ssDNA synthesis and its profound implications in genetic research as well as its advanced applications.
Research Overview
Summary of Key Findings
The synthesis of single-stranded DNA relies on a series of biochemical reactions that can be fine-tuned for specific purposes. Key findings from recent studies reveal:
- ssDNA is synthesized using enzymes such as DNA polymerases and ligases.
- The process is influenced by temperature, buffer conditions, and nucleotide availability.
- Recent technological advancements have improved the accuracy and yield of ssDNA synthesis.
These findings underscore the importance of ssDNA in various biotechnological applications and its role in advancing genetic research.
Background and Context
Single-stranded DNA has gained considerable attention in the last few decades, due to its unique properties. Traditional DNA synthesis methods typically yield double-stranded DNA. However, ssDNA is often required in specific applications, such as PCR amplification and hybridization assays. The ability to produce ssDNA efficiently can significantly impact fields such as genomics, molecular diagnostics, and personalized medicine.
With growing interest in genetically engineered organisms and gene therapies, a thorough understanding of ssDNA synthesis becomes paramount.
Methodology
Experimental Design
Understanding ssDNA synthesis involves multiple approaches. Researchers often utilize both in vitro and in vivo methodologies, tailoring their design based on the application. For example, In vitro synthesis focuses on enzyme-catalyzed reactions, while in vivo methods leverage cellular machinery for greater complexity and production levels.
Data Collection Techniques
Data is collected through various techniques including:
- Spectrophotometry: This technique measures the concentration of ssDNA, providing insight into synthesis efficiency.
- Gel Electrophoresis: This method can determine the size and quality of the synthesized ssDNA.
- Next-Generation Sequencing: This advanced technique enables comprehensive analysis of the synthesized DNA, ensuring accuracy and confirming the integrity of the product.
These methods highlight the importance of accurate data collection in optimizing ssDNA synthesis processes.
"The synthesis of ssDNA is not just a technical challenge; it embodies the complexities of molecular biology that can lead to groundbreaking advances in health and science."
In summary, this article will present a detailed examination of ssDNA synthesis, uncovering the mechanisms, applications, and recent advances that shape the future of genetic research. This exploration aims to provide a comprehensive guide for students, researchers, educators, and professionals engaged in the field.
Understanding SSDNA
Single-stranded DNA (ssDNA) plays a crucial role in various biological and technological processes. Understanding ssDNA is imperative, as it serves as a fundamental component in genetic studies, diagnostics, and synthetic biology. The exploration of ssDNA helps clarify its distinct functions, properties, and relevance compared to its double-stranded counterpart.
Recognizing ssDNA accurately aids in comprehending associated mechanisms for synthesis and applications. The ability to generate and manipulate ssDNA has profound implications for research, bringing enhancements in gene therapy, molecular diagnostics, and synthetic biology. In this article, we will unfold the nuances of ssDNA, evaluate its biochemical foundations, and delve into its synthesis mechanisms and applications.
Definition and Nature of SSDNA
Single-stranded DNA (ssDNA) is a form of nucleic acid that consists of a single strand of nucleotide sequences. Unlike double-stranded DNA, which features two complementary strands wound around each other, ssDNA exists independently. Its structure allows it to fold upon itself, creating secondary formations, but it remains unpaired with another strand.
The nature of ssDNA makes it versatile and functional in various biological processes. For instance, ssDNA is essential during DNA replication, where it serves as a template for synthesizing complementary strands. Likewise, ssDNA is employed in techniques like Polymerase Chain Reaction (PCR) and plays a critical role in cloning and gene manipulation. Understanding ssDNA partners with knowing its interactions with proteins and other molecular players, which is vital for discerning its full scope in biotechnology.
Comparison with Double-Stranded DNA
The contrast between ssDNA and double-stranded DNA (dsDNA) lies primarily in their structural organizations and biological functions.
- Structure: ssDNA consists of a single strand of nucleotides, whereas dsDNA comprises two complementary strands. The double helix structure of dsDNA contributes to its stability and protective function, which is not present in ssDNA.
- Functionality: ssDNA is mainly involved in processes that require accessibility, such as replication and transcriptions. In contrast, dsDNA serves as the long-term storage form of genetic information. The stability of dsDNA makes it suitable for long-term information retention, while ssDNA's flexibility allows quicker access to genetic code for transcription and translation.
- Applications: ssDNA has significant applications in molecular diagnostics and therapeutic contexts, whereas dsDNA is often utilized in genotyping, sequencing, and structural studies.
Understanding these differences is essential as they influence various techniques used in research and industry. The unique properties of ssDNA allow scientists to leverage its characteristics for innovative approaches and applications in genetic research and biotechnology.
Biochemical Foundations
Understanding the biochemical foundations of single-stranded DNA (ssDNA) synthesis is essential for comprehending its role in molecular biology and its numerous applications in research and industry. These foundations offer insight into the structure and function of nucleic acids and the enzymes that facilitate their synthesis. Grasping these concepts will enhance our understanding of ssDNA's significance in various biotechnological arenas.
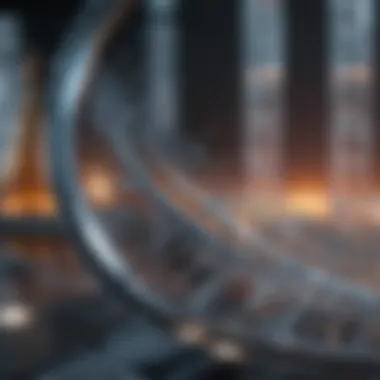
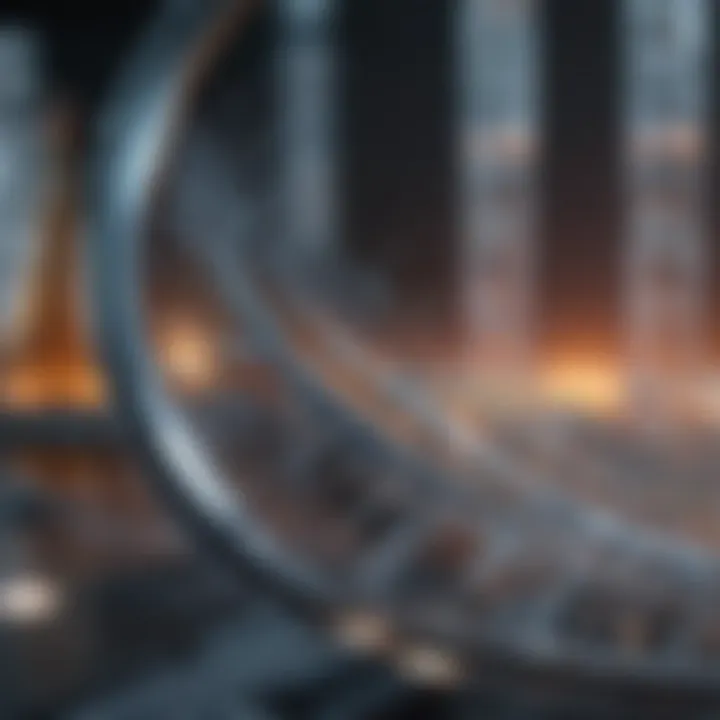
Nucleic Acid Structure
Nucleic acids, comprising both DNA and RNA, serve as the fundamental building blocks of genetic material. These macromolecules are constructed from long chains of nucleotides, each consisting of a sugar, a phosphate group, and a nitrogenous base. In ssDNA, the particular sequence of nucleotides carries the necessary information for coding genetic traits. The characteristic features of ssDNA include its linear arrangement and the presence of four primary bases: adenine, thymine, cytosine, and guanine.
Unlike double-stranded DNA, which has complementary strands that pair through hydrogen bonds, ssDNAβs unpaired state allows for unique interactions with proteins and other nucleic acids. This unpaired characteristic is crucial for functions such as replication and transcription, which often necessitate the exposure of nucleotide sequences. Moreover, the structural configuration of ssDNA must be noted as it influences its stability and interactions within biological systems.
The Role of Enzymes in Synthesis
Enzymes play a pivotal role in the synthesis of ssDNA. They facilitate the biochemical reactions required to construct ssDNA from nucleotides, ensuring that the process is efficient and accurate. One key enzyme involved in this process is DNA polymerase. It is responsible for adding nucleotides to the growing ssDNA strand during replication.
Other enzymes, such as ligases and exonucleases, are also vital. Ligases join together DNA fragments, while exonucleases can determine the fidelity of the synthesis by removing incorrect nucleotides.
"The role of enzymes cannot be overstated; they are the facilitators that make ssDNA synthesis feasible in a controlled and reproducible manner."
In summary, a sound understanding of the biochemical foundations that underlie ssDNA synthesis is crucial. The structure of nucleic acids and the role of enzymes collectively highlights the complexity and efficiency of biological systems, laying the groundwork for further exploration into the mechanisms and applications of ssDNA.
Mechanisms of SSDNA Synthesis
Understanding the mechanisms of SSDNA synthesis is crucial for researchers and professionals engaged in genetics and molecular biology. SSDNA plays an essential role in various applications, from diagnostics to therapeutic methods. Hence, knowing how it's synthesized can inform the choice of techniques used in specific contexts. It enhances efficiency, opens up new pathways for research, and guides improvements in technology. In this section, we will explore the different pathways involved, the processes of priming and extension, and the factors that influence the yield of synthesis.
Natural vs. Synthetic Pathways
The synthesis of SSDNA occurs through two primary pathways: natural and synthetic. Natural pathways primarily involve cellular processes where various enzymes catalyze the formation of single-stranded DNA as part of DNA replication and repair mechanisms. For instance, viral DNA replication often results in single strands, showcasing natural biological mechanisms at work.
On the other hand, synthetic pathways involve laboratory techniques designed to create SSDNA for specific research or industrial purposes. One common method is through chemical synthesis, which uses automated synthesizers to produce sequences as desired. The advantage of synthetic methods is the ability to create highly specific sequences, which can be customized for various applications.
Both methods have their benefits and limitations. Natural synthesis can yield higher fidelity and authenticity, stemming from complex biological processes. However, synthetic pathways allow for precision and flexibility to design novel sequences not found in nature. Evaluating these pathways can help researchers decide which synthesis method best suits their needs, whether for diagnostics, research, or therapeutic applications.
Priming and Extension Processes
The priming and extension stages are critical in any SSDNA synthesis process. Priming is the initial step wherein a short segment of nucleic acid is synthesized. This segment acts as the starting point for further elongation. The primer must be complementary to the SSDNA template, ensuring accurate synthesis. In PCR, for instance, primers flank the region of interest, initiating DNA replication during the extension phase.
During the extension phase, DNA polymerase enzymes add nucleotides to the growing strand, extending the SSDNA. This process is influenced by several factors, including temperature and enzyme concentration. Reaction conditions must be established carefully to optimize yields and accuracy. Additionally, the type of polymerase used can have substantial implications on both the rate and fidelity of the synthesis, making these choices vital for successful outcomes.
Factors Influencing Synthesis Yield
Multiple factors impact the yield of SSDNA synthesis. A key element is the quality of the starting materials, such as nucleotides, enzymes, and templates. Impurities or degradation can reduce the efficiency significantly. For synthetic methods, the design of oligonucleotides also plays an important role. Sequences that are highly repetitive or contain secondary structures may lead to lower yields due to difficulties in amplification or synthesis errors.
Temperature control during synthesis is another crucial consideration. High or low temperatures can lead to undesirable outcomes, such as denaturation or non-specific binding. Additionally, the ionic strength of the solution and the presence of additives can facilitate or hinder the synthesis, showcasing the importance of optimizing reaction conditions.
Quality assurance steps, including monitoring synthesis through gel electrophoresis or sequencing, provide feedback on the success of the synthesis process. By comprehensively understanding these factors, researchers can innovate methods that improve the quality and quantity of SSDNA produced.
"The mechanisms underlying SSDNA synthesis are pivotal in enhancing the efficiency and specificity of biotechnological applications."
In summary, the mechanisms of SSDNA synthesis reveal essential insights into its natural and synthetic processes. Recognizing the nuances of priming, extension, and influencing factors provides a solid foundation for tackling challenges in genetic research and applications.
Technical Approaches to SSDNA Synthesis
The field of SSDNA synthesis relies heavily on various technical methods. Each approach has its own unique benefits, functional strengths, and considerations. Understanding these methods is essential for anyone working with or studying ssDNA. Each technique offers specific pathways to synthesize and manipulate single-stranded DNA, and choosing the right method can determine the quality and efficiency of ssDNA generated.
Polymerase Chain Reaction (PCR)
Polymerase Chain Reaction, commonly known as PCR, is one of the most widely used techniques for the amplification of DNA segments. Its relevance in ssDNA synthesis cannot be overstated. PCR allows scientists to generate large amounts of DNA from a minuscule template by utilizing heat-stable DNA polymerases, such as Taq polymerase. The process consists of three main steps: denaturation, annealing, and extension.
- Denaturation: The double-stranded DNA melts open to form two single strands.
- Annealing: Primers attach to the ssDNA templates, necessary to initiate synthesis.
- Extension: DNA polymerase synthesizes new strands, producing a new double-stranded DNA.
Although PCR is remarkable for its efficiency, it is essential to manage conditions properly. Suboptimal temperatures can lead to non-specific binding, affecting the yield and purity of the synthesized ssDNA. Thus, a carefully optimized protocol is fundamental to the successful application of PCR in synthesizing ssDNA.
Important Note: PCR is instrumental in various applications, from genetic research to cloning and forensic analysis, making it an invaluable tool in molecular biology.
Ligation-Mediated Techniques
Ligation-mediated techniques offer another approach for constructing ssDNA. These methods primarily involve the joining of two or more DNA fragments through a ligase enzyme. This technique allows researchers to create longer, more complex ssDNA sequences, which can be essential when assembling constructs for gene editing or synthetic biology.
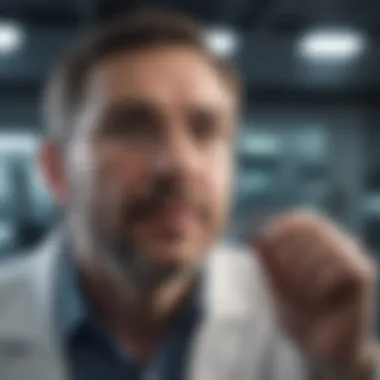
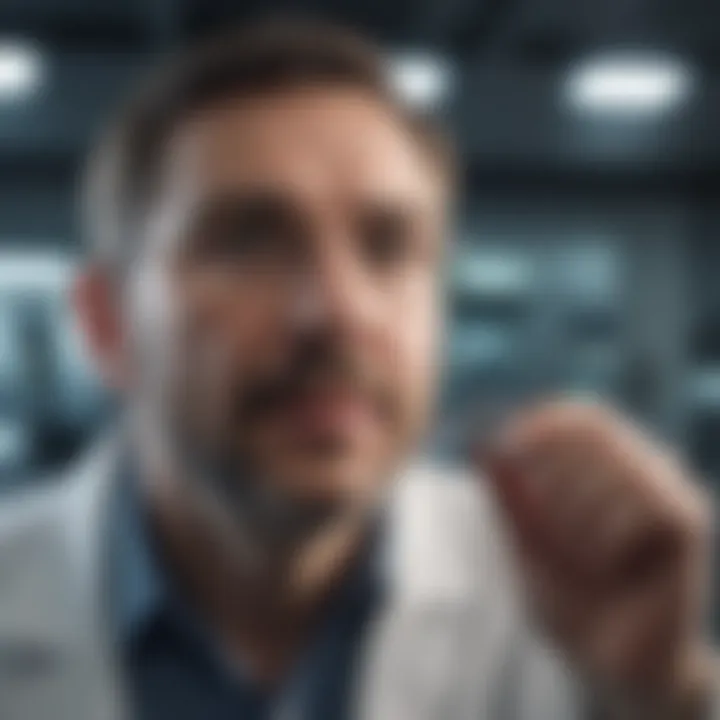
The ligation process typically consists of two steps:
- Preparation of DNA Fragments: Each fragment must have compatible ends, often facilitated by restriction enzymes.
- Ligation: DNA ligase enzyme links the fragments together, forming a continuous ssDNA strand.
One major benefit of this approach is its ability to generate specific sequences tailored for distinct experimental needs. However, ligation efficiency can vary, and producing high-quality ssDNA may require purification steps to remove unligated fragments and enzymes post-reaction.
Solid-Phase Synthesis Approaches
Solid-phase synthesis is a powerful technique for creating ssDNA and has gained considerable traction in both academic and commercial domains. In this method, ssDNA is constructed on a solid support using a stepwise assembly approach that allows for precise control over the sequence and length of the DNA.
Key elements in this method include:
- Stepwise Addition: Nucleotides are sequentially added to a growing chain while anchored to the solid surface.
- Cleavage: After synthesis, the ssDNA can be cleaved from the solid support, yielding a pure product.
This approach permits the synthesis of overwhelmingly diverse and long ssDNA sequences, which can be utilized in various applications from probe design to therapeutic development. Nonetheless, high initial costs and the need for specialized equipment are common challenges associated with solid-phase synthesis.
Applications of SSDNA
The applications of single-stranded DNA (ssDNA) are extensive, influencing diverse fields such as diagnostics, therapeutics, and synthetic biology. Each application utilizes the unique properties of ssDNA to achieve specific outcomes. Understanding these applications provides insight into both the value of ssDNA in research and its potential impact on future innovations.
Role in Molecular Diagnostics
Molecular diagnostics rely heavily on ssDNA due to its capability for specific binding and hybridization. The sensitive detection of nucleic acid sequences is paramount in clinical settings. For example, ssDNA probes are used in techniques such as polymerase chain reaction (PCR) and fluorescence in situ hybridization (FISH). These methods allow for the rapid identification of pathogens and genetic anomalies.
The use of ssDNA enhances specificity in hybridization assays. This means that ssDNA can effectively distinguish between closely related sequences, reducing the likelihood of false positives. In infectious diseases, timely detection improves patient outcomes through quicker treatment decisions. In oncology, ssDNA can identify mutations associated with various cancers, guiding personalized medicine approaches.
"The sensitivity and specificity of ssDNA make it indispensable for advancing molecular diagnostics in healthcare."
Use in Therapeutics and Gene Therapy
ssDNA also plays a crucial role in therapeutics, particularly in gene therapy. It can be used to deliver therapeutic genes into cells. Here, ssDNA acts as a vector to carry the desired genetic material. This provides a novel method for treating genetic disorders by enabling the correction of faulty genes.
In situations like CRISPR-Cas9 gene editing, ssDNA is utilized as a repair template. This allows for precise edits in the genome of living organisms. The incorporation of ssDNA enhances the efficiency of gene editing processes, making it a vital component in developing therapies for conditions like cystic fibrosis and muscular dystrophy.
Applications in Synthetic Biology
In synthetic biology, ssDNA serves as a foundational tool for constructing biological systems and organisms. Researchers manipulate ssDNA to design new genetic circuits that can perform specific functions within a cell. This process has applications in biofuel production, environmental monitoring, and the development of biosensors.
There is also significant interest in the use of ssDNA for the creation of novel biomolecules. For example, the synthesis of peptide nucleic acids (PNAs) relies on ssDNA as a building block. These PNAs have potential applications in various fields, including advanced diagnostics and therapeutic development.
Furthermore, ssDNA plays a role in creating organisms with tailored characteristics, suitable for biochemical productions or environmental remediation.
In summary, the applications of ssDNA are diverse and impactful. Its role in molecular diagnostics enhances detection capabilities, while contributions to therapeutics revolutionize treatment options. Finally, in synthetic biology, ssDNA opens the door to innovative solutions for complex biological challenges.
Recent Advances in SSDNA Synthesis
The field of single-stranded DNA (ssDNA) synthesis has witnessed significant advancements that have revolutionized both theoretical understanding and practical applications. These recent developments are critical, as they enhance not only the efficiency of ssDNA production but also its applicability in various scientific domains. Understanding these advancements offers insight into how they can potentially shape future research and innovations.
Innovations in Synthesis Techniques
New synthesis techniques are continuously emerging, offering improved methods for the production of ssDNA. Innovations such as enzymatic synthesis, where polymerases are optimized to enhance speed and accuracy, have emerged as game changers. These advancements allow scientists to engineer organisms capable of producing ssDNA more efficiently, reducing the time and cost associated with traditional production methods.
Another area of innovation is the development of microfluidic devices that enable high-throughput synthesis. These devices allow for rapid iterations and adjustments, leading to a more efficient synthesis process. By miniaturizing the synthesis environment, researchers can obtain precise control over conditions such as temperature and reagent concentration, which are crucial for optimal yield.
Additionally, new software tools assist in designing ssDNA sequences for applications in diagnostics and therapeutics. These tools can predict secondary structures, making it easier for researchers to create effective ssDNA for specific applications.
Improvements in Accuracy and Efficiency
In the realm of ssDNA synthesis, accuracy and efficiency are paramount. Recent advances have focused on overcoming previous limitations that often resulted in low yield and high error rates. Improved enzymatic formulations now provide higher fidelity in strand synthesis, ensuring that the resultant ssDNA is not only pure but also functionally relevant for its intended applications.
Furthermore, advancements in purification techniques are enhancing the quality of synthesized ssDNA. Methods such as affinity chromatography and gel electrophoresis have been refined to ensure that the final product consists predominantly of the desired single-stranded molecules, minimizing contamination.
Recent developments in controlling synthesis conditions have also contributed to increased yields. Parameters such as pH and reagent concentrations can now be precisely adjusted in real-time, enhancing both output and purity.
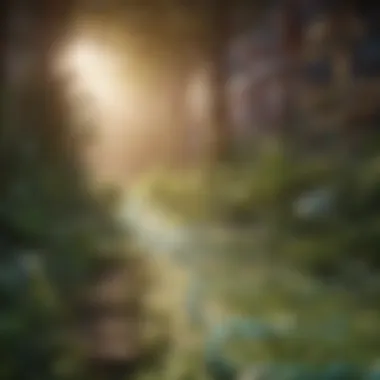
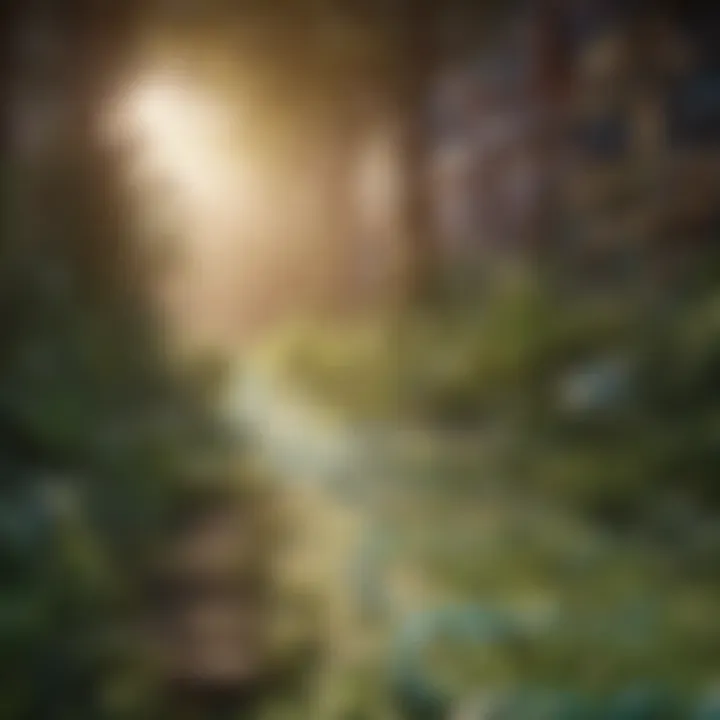
Overall, these improvements lead to notable benefits like reduced costs and increased scalability, making it more feasible for laboratories to conduct extensive ssDNA synthesis for various purposes.
Challenges in SSDNA Synthesis
The challenges faced in the synthesis of single-stranded DNA (ssDNA) are significant. Understanding these challenges is crucial for students, researchers, and professionals in the field of molecular biology. These challenges can hinder progress in various applications, including diagnostics and genetic engineering. Identifying technical limitations and cost implications enables researchers to develop better strategies.
Technical Limitations
Technical limitations in ssDNA synthesis can arise from several factors. One primary concern involves the inherent instability of single-stranded molecules. ssDNA is prone to degradation by various nucleases and environmental factors. This instability can result in lower yields of usable product.
Moreover, many synthesis methods, such as polymerase chain reaction (PCR) or solid-phase synthesis, can introduce errors. Issues like misincorporation of nucleotides can occur, leading to sequences that are not biologically relevant.
Another limitation arises from the length of ssDNA that can be synthesized. Longer strands often present difficulties in maintaining stability and integrity during the synthesis process. Techniques capable of producing longer ssDNA are still in development and may not yield optimal results. Thus, the synthesis of longer ssDNA continues to be a technical challenge.
Cost Implications of High-Quality Synthesis
Cost is a significant factor when it comes to high-quality ssDNA synthesis. The demand for high accuracy demands advances in technology, which can elevate costs. High-quality nucleotides and enzymes may be required. These are essential for achieving desired synthesis results but can increase the overall budget.
Furthermore, specialized equipment or reagents are sometimes necessary. This may include advanced thermal cyclers for PCR or automated synthesizers for solid-phase synthesis. Their acquisition and maintenance add yet another layer of expense.
Researchers must also consider the expense of optimizing conditions for each specific synthesis case. This involves experimentation and refinement, which can consume valuable time and resources. Therefore, balancing the quality of ssDNA produced and the associated costs is an ongoing area of concern.
"Research in ssDNA synthesis must address both technical limitations and cost implications for broader applications."
Future Directions in Research
The field of single-stranded DNA (ssDNA) synthesis is advancing rapidly, driven by the need for more efficient and versatile methods. Understanding future directions in this area is crucial. It holds significant implications not just for theoretical research but also for real-world applications in medicine, diagnostics, and biotechnology. The exploration of new enzymatic pathways, enhancements in scalability, and the potential for expanded applications are vital components of this development.
Exploring New Enzymatic Pathways
One area that stands to benefit from exploration is the discovery of novel enzymatic pathways. Traditional methods of ssDNA synthesis often rely heavily on established enzymes such as Taq polymerase. However, the increasing availability of various enzymes sourced from extremophiles or engineered proteins offers new avenues. These enzymes can provide greater efficiency and specificity during synthesis.
Research into these new pathways can significantly alter synthesis strategies. Enzymes that exhibit robust performance under various conditions may provide options for synthesizing ssDNA in challenging environments. This not only enhances productivity but also reduces waste and the reliance on harsh chemicals. Moreover, insights gained from studying these enzymes can lead to improvements in synthetic biology, where precision is paramount.
Enhancing Scalability of Techniques
Scalability poses another challenge in ssDNA synthesis. Current methods often function effectively on a small scale but falter when scaled up for industrial applications. The ability to produce large quantities of ssDNA while maintaining quality is pivotal for commercial ventures. By focusing on developments that enhance scalability, researchers can bridge the gap between laboratory and industrial synthesis.
Efforts to improve scalability may involve optimizing existing protocols or developing entirely new methodologies. For example, shifts towards automated systems and integration with high-throughput screening can streamline the production process. These enhancements can lead to economic viability, making large-scale synthesis more feasible and attractive for industries such as pharmaceuticals and genetic engineering.
Potential for Expanded Applications
The potential applications of ssDNA are vast and varied. As research continues, the scope of where ssDNA can be effectively used keeps expanding. Beyond current applications in molecular diagnostics and therapeutics, there is possibility for integration into new areas.
For instance, ssDNA can play a role in next-generation sequencing, providing a more flexible medium for data collection and analysis. Additionally, its utility in developing advanced biosensors could lead to novel diagnostic tools. As ssDNA synthesis methods improve, so too will the ability to address emerging needs in health care, environmental monitoring, and even data storage.
Ultimately, understanding these future directions in ssDNA synthesis will pave the way for transformative advancements in a variety of scientific fields.
"The continuous evolution of ssDNA synthesis techniques holds promise not only for enhancing existing applications but also for uncovering entirely new avenues for research and industrial applications."
Research in ssDNA synthesis is at a pivotal point, and diligent exploration in the highlighted areas can lead to significant breakthroughs with wide-reaching impact.
The End
The conclusion of this article serves to synthesize the varied aspects of single-stranded DNA (ssDNA) synthesis explored in prior sections. The discussion sheds light on critical components such as mechanisms, applications, and advances in technology. This is key for understanding how ssDNA fits into the wider landscape of genetic research.
Summary of Key Points
- Understanding ssDNA is foundational to recognizing its role in advanced molecular diagnostics and gene therapy.
- Biochemical foundations underpin all processes, highlighting the significance of enzymes in synthesis.
- Mechanisms of synthesis differ in natural and synthetic pathways, which can significantly influence yield and quality.
- Technical approaches such as Polymerase Chain Reaction (PCR) and solid-phase synthesis have evolved, leading to greater precision and efficiency.
- Applications span multiple fields, proving integral in molecular diagnostics, therapeutics, and synthetic biology.
- Recent advances have focused on enhancing accuracy and throughput, positioning ssDNA technology at the forefront of genetic innovation.
- Challenges still exist, primarily surrounding technical limitations and cost factors.
- Future directions in research present possibilities for new enzymatic pathways and expanded applications, indicating ongoing relevance and importance of ssDNA.
In summary, ssDNA synthesis represents a dynamic intersection of technology and biological understanding. This article elucidates these connections, promoting a comprehensive outlook on its current and future impact.
Implications for the Scientific Community
The implications of ssDNA synthesis and its advancements are significant for the scientific community. Particularly in molecular biology and genetics, researchers can leverage the insights presented to refine methodologies and improve experimental outcomes. Furthermore, as ssDNA plays a crucial role in diagnostics and therapeutics, advancements can have real-world benefits, streamlining processes and enhancing patient outcomes.
Additionally, an understanding of ssDNA can inspire innovative research initiatives. By exploring unexplored enzymatic pathways and developing cost-effective synthesis methods, researchers may achieve higher scalability and wider application of ssDNA in synthetic biology. This also implies a need for interdisciplinary collaboration, fostering a community that can bridge gaps between theoretical knowledge and practical application.
"The horizon of single-stranded DNA research is expansive, promising substantial contributions to future scientific inquiries and health advancements."