Understanding the Structure of Chemical Compounds
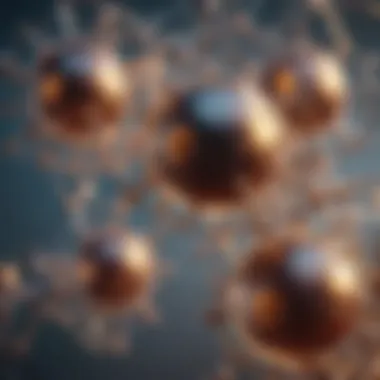
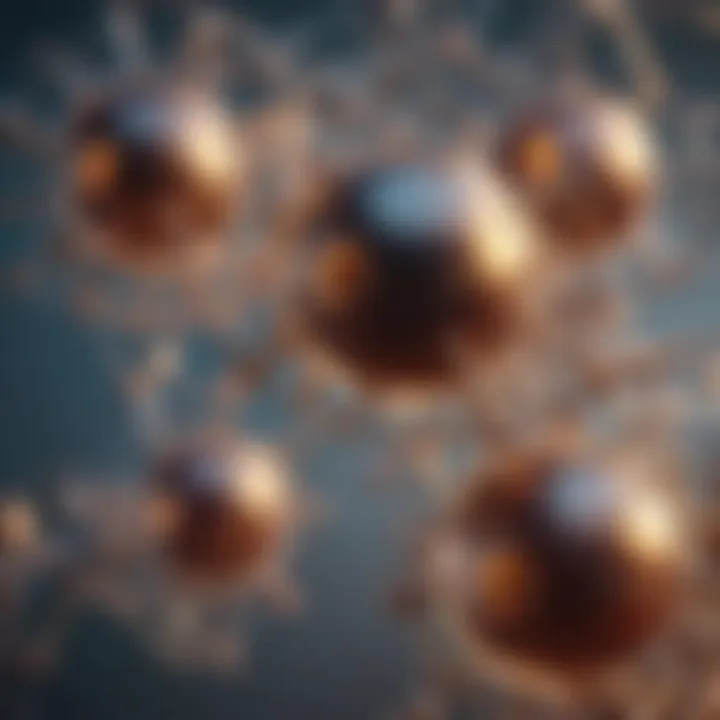
Intro
Understanding the structure of chemical compounds is fundamental to the field of chemistry. The arrangement of atoms within these compounds can determine their properties, behavior, and reactivity. This article aims to provide a comprehensive examination of structural chemistry, focusing on how molecular architecture influences various chemical processes. By exploring concepts such as bonding, molecular geometry, and functional groups, we can gain insights into the underlying principles that govern chemical systems.
Research Overview
Summary of Key Findings
The research indicates that the geometry of molecules profoundly affects their reactivity and properties. Molecular structures, including ionic, covalent, and metallic compounds, exhibit distinct characteristics that can be observed in reactions and interactions.
Furthermore, advanced facets such as stereochemistry and resonance structures highlight the complexity of molecular behavior. These elements are vital in predicting physical and chemical properties for various applications, such as drug design and material development.
Background and Context
The study of chemical structures dates back to early chemistry, where the arrangement of elements was first recognized as a decisive factor in understanding chemical reactions. With the advent of modern techniques like X-ray crystallography and spectroscopy, we now have the ability to analyze molecular structures in greater detail than ever before. This advancement facilitates deeper insights into how modifications in molecular architecture can lead to varying chemical behaviors.
Methodology
Experimental Design
The methodology encompasses both theoretical analyses and experimental investigations. Researchers utilize computational chemistry methods to model structures and predict outcomes. Laboratory experiments further solidify these findings through empirical validation.
Data Collection Techniques
Data collection often involves a mix of qualitative and quantitative approaches. Techniques such as X-ray diffraction and nuclear magnetic resonance spectroscopy provide critical insights into the arrangement of atoms and the properties of molecules. These techniques are essential for analyzing both pure compounds and complex mixtures, thus offering a comprehensive understanding of chemical structure and function.
"The ability to visualize molecular structures is crucial for understanding their behavior in chemical reactions."
Preamble to Chemical Structures
Understanding the foundational concepts of chemical structures is vital in the field of chemistry. Chemical structures determine the properties, reactivity, and functionality of compounds. By studying these structures, one gains insights into how atoms bond, how molecules behave under different conditions, and how they interact with one another. This comprehension is essential not just for students or researchers but also for professionals working in various fields such as pharmaceuticals, materials science, and environmental science.
Defining Chemical Structure
Chemical structure refers to the arrangement of atoms within a molecule. This includes how atoms are connected, the shape of the molecule, and the bonds that form between atoms. There are several ways to depict chemical structures, including molecular formulas, Lewis structures, and three-dimensional models.
For example, the molecular formula of water is HβO, indicating two hydrogen atoms bonded to one oxygen atom. However, the Lewis structure emphasizes the electron pairs involved in bonding, showing the interactions more clearly. Understanding these concepts is crucial for predicting the behavior of molecules in chemical reactions, as the arrangement of atoms directly influences their interactions and transformations.
Importance of Structure in Chemistry
The significance of chemical structure in chemistry cannot be underestimated. The structure influences many aspects of chemical compounds, including:
- Physical properties: The boiling and melting points, solubility, and density depend heavily on molecular structure.
- Chemical reactivity: The way molecules react with each other is determined by their structures. For example, functional groups within organic compounds largely dictate how they will undergo reactions.
- Biological activity: In fields like medicinal chemistry, the efficacy of a drug often correlates with its molecular structure. Small changes in structure can lead to vastly different biological responses.
In summary, the exploration of chemical structures provides a base knowledge necessary for deeper study in chemistry. By understanding the various factors that contribute to the characteristics and functions of molecules, one can make informed predictions about their behavior in a variety of contexts.
Types of Chemical Bonds
Chemical bonds serve as the construction elements of molecules and compounds. They establish the connections between atoms and determine the properties and behavior of substances. Understanding the types of chemical bonds is crucial for dissecting the complexity of chemical interactions. This section discusses three primary types of chemical bonds: ionic, covalent, and metallic. Each bond type has its distinct characteristics, benefits, and implications in both theoretical and applied chemistry.
Ionic Bonds
Ionic bonds are formed through the transfer of electrons between atoms. Typically, this occurs between atoms that have significantly different electronegativities, such as sodium and chlorine. In this bond, one atom loses one or more electrons, becoming a positively charged ion, while the other atom gains those electrons, turning into a negatively charged ion. This electrostatic attraction between the oppositely charged ions results in a strong ionic bond.
Ionic bonds are key in determining the properties of ionic compounds. These compounds often have high melting and boiling points due to the strong interactions among ions. Furthermore, when dissolved in water, ionic compounds tend to dissociate into their constituent ions, which allows them to conduct electricity.
Key things to note about ionic bonds include:
- Formation typically involves metals and nonmetals.
- Their solid-state structure is arranged in a crystal lattice.
- They are generally soluble in polar solvents such as water.
Covalent Bonds
Covalent bonds form when two atoms share pairs of electrons. This type of bond typically exists between non-metal atoms with similar electronegativities, allowing them to achieve a stable electron configuration. The shared electrons create a balance between the attractive and repulsive forces, leading to a bond that can be either single, double, or even triple, depending on the number of shared electron pairs.
Covalent bonds play a central role in organic chemistry and the structure of biological molecules. For example, water consists of polar covalent bonds, where electrons are shared unevenly between oxygen and hydrogen atoms. This uneven distribution of charge influences molecular properties, such as polarity.
Some important facts to highlight regarding covalent bonds:
- They can form between identical atoms (e.g., in Oβ) or different atoms (e.g., in HβO).
- Covalent compounds generally have lower melting and boiling points compared to ionic compounds.
- They can form complex structures, influencing the reactivity and properties of molecules.
Metallic Bonds
Metallic bonds arise from the attraction between positively charged metal ions and delocalized electrons in a metal lattice. This unique bonding type enables metals to exhibit characteristic properties, such as electrical conductivity, malleability, and ductility. The delocalized nature of electrons allows them to move freely, making it possible for metals to conduct electricity efficiently.
These bonds are not only crucial for understanding the behavior of metals but also provide insight into the fundamentals of alloy development. Metallic bonding plays a significant role in the functionality of materials, including those used in construction and technology.
Key characteristics of metallic bonds include:
- Metals can change shape without breaking due to the malleability created by metallic bonds.
- They typically feature a shiny appearance, known as luster.
- High thermal and electrical conductivity are common, due to the mobility of electrons.
Molecular Geometry
Molecular geometry refers to the three-dimensional arrangement of atoms within a molecule. This concept is fundamental in understanding how molecules interact with one another and with varying substances. The geometry of a molecule is affected by the types of bonds between atoms and the presence of lone pairs of electrons. These arrangements not only determine the shape of molecules but also their properties and reactivity.
In structural chemistry, molecular geometry helps predict physical properties such as boiling points, melting points, and solubility. For example, the polar nature of water arises from its bent shape, which influences its interactions with other molecules. Thus, knowing the geometry can aid in anticipating how a substance behaves in different environments.
There are several key considerations when discussing molecular geometry:
- Bond Angles: The angles between adjacent bonds in a molecule can greatly affect its shape and properties.
- Steric Effects: The spatial arrangement of atoms can create varying levels of steric hindrance, impacting reactions and stability.
- Electronic Repulsion: Lone pairs of electrons repel bonding pairs, altering the molecular shape.
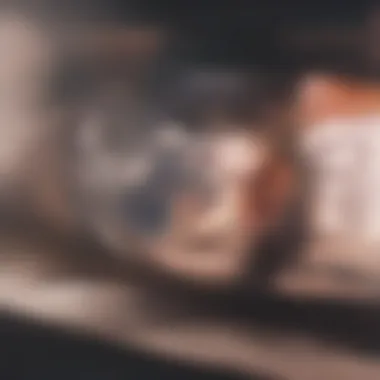
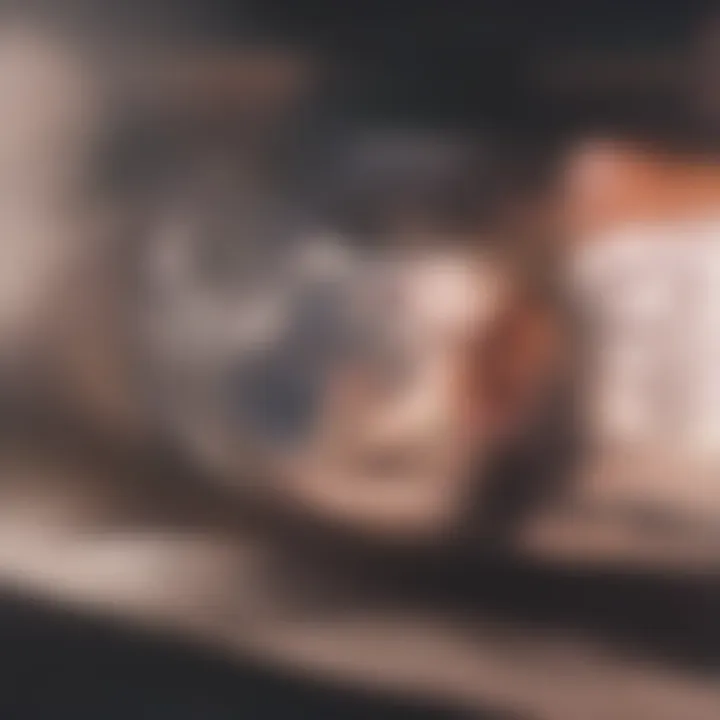
Understanding molecular geometry is vital for students and researchers in chemistry as it provides clarity on how molecular interactions play a role in various reactions in the field.
VSEPR Theory
The Valence Shell Electron Pair Repulsion (VSEPR) theory is a key model used to predict the geometry of molecules. This theory is based on the principle that electron pairs in the valence shell of an atom repel each other, and thus, arrange themselves to minimize this repulsion. It categorizes molecular shapes based on the number of bonding pairs and lone pairs around the central atom:
- Linear Geometry: This occurs when there are two bonding pairs with no lone pairs, like in carbon dioxide (COβ).
- Trigonal Planar Geometry: Three bonding pairs and no lone pairs lead to a flat triangular shape, such as in boron trifluoride (BFβ).
- Tetrahedral Geometry: Four bonding pairs create a three-dimensional shape, exemplified by methane (CHβ).
VSEPR theory allows chemists to predict and visualize molecular shapes quickly, making it an essential tool in structural chemistry.
Hybridization
Hybridization is another crucial concept in understanding the geometry of molecules. This process involves the mixing of atomic orbitals to form new hybrid orbitals. These hybrid orbitals can blend different types of orbitals, such as s and p orbitals, which allows for the formation of more complex bonding patterns.
Different types of hybridization result in distinct molecular geometries:
- sp Hybridization: Two atomic orbitals mix to form two equivalent sp hybrid orbitals, producing a linear geometry, as seen in acetylene (CβHβ).
- spΒ² Hybridization: Three orbitals combine to form three spΒ² hybrid orbitals, giving rise to trigonal planar shapes, like in ethene (CβHβ).
- spΒ³ Hybridization: The mixing of one s and three p orbitals results in four equivalent spΒ³ hybrid orbitals, which characterize tetrahedral molecules such as methane.
Hybridization not only explains the geometry of a molecule but also its bonding properties, influencing reactivity and stability.
"Understanding molecular geometry, including the implications of VSEPR theory and hybridization, is essential for anyone engaged in advanced chemical research."
Through these principles, structural chemistry expands its grasp on predicting and explaining the behavior of a multitude of substances in various contexts.
Functional Groups in Organic Chemistry
Functional groups are specific groups of atoms within molecules that have distinct characteristics and chemical properties. They play a crucial role in the structure and reactivity of organic compounds. Understanding functional groups is essential for analyzing organic chemistry since they dictate how a compound behaves in chemical reactions and their overall properties.
The study of functional groups provides students, researchers, and professionals with a framework to classify and predict reactions among various organic compounds. This knowledge allows chemists to design novel substances with customized properties, enhancing applications in pharmaceuticals, materials science, and other fields.
Overview of Functional Groups
Functional groups can be understood as the building blocks of larger organic molecules. Common functional groups include hydroxyl groups (-OH), carboxyl groups (-COOH), amines (-NHβ), and carbonyl groups (C=O). Each of these groups imparts unique properties to the compounds in which they are present.
- Hydroxyl group: Found in alcohols, this group makes molecules polar and increases their solubility in water.
- Carboxyl group: Present in carboxylic acids, it brings acidity to the compound.
- Amines: These groups are integral in forming amines, which can act as bases in reactions.
- Carbonyl group: Essential in ketones and aldehydes, influencing reactivity and molecular structure.
Identifying functional groups in organic molecules aids in predicting their chemical behavior while guiding the synthesis of pharmaceuticals and biochemical agents. As a result, these groups act as a shorthand for describing the chemistry of compounds.
Reactivity and Functionality
The presence of functional groups directly influences the reactivity patterns of organic molecules. Different functional groups can lead to various reaction pathways and outcomes, therefore profoundly affecting the properties of substances. The reactivity often correlates with the electronegativity of the atoms in the functional group and their arrangement within the molecule.
For instance, alcohols can undergo oxidation reactions to yield ketones or aldehydes, while carboxylic acids can react with bases to form salts and water. Moreover, functional groups often serve as sites for nucleophilic or electrophilic attack in organic reactions, fundamentally driving the course of chemical transformations.
Several factors determine the significance of functional groups:
- Position within the molecule: This can alter the properties and reactivity.
- Stability of the molecule: Functional groups can stabilize or destabilize intermediates in reactions.
- Intermolecular interactions: Groups like -OH or -NHβ enhance hydrogen bonding, impacting solubility and boiling points.
"Understanding functional groups is like having a map in the chemical landscape. They guide reactions and help predict outcomes."
In summary, functional groups are pivotal to the study of organic chemistry. They help in understanding molecular identity, assessing reactivity, and predicting behavior in numerous chemical contexts. This knowledge is instrumental for those engaged in various fields, particularly when creating new compounds or investigating chemical processes.
By grasping the role and significance of functional groups, one gains insights into the structural foundations that underpin the diverse world of organic compounds.
Resonance Structures
Understanding resonance structures is pivotal in structural chemistry. Resonance contributes to explaining the stability and reactivity of chemical compounds. It represents a way to illustrate the delocalization of electrons, which plays a crucial role in how molecular structures behave. When molecules can exist in multiple forms, known as resonance forms, their actual structure is a hybrid of these forms. This concept is essential for researchers and professionals as it offers insights into molecular behavior.
Concept of Resonance
Resonance is characterized by the presence of two or more valid Lewis structures for a single molecule. Each structure is an approximation that depicts how electrons are distributed in the molecule. These alternative representations help in understanding the actual distribution of electrons, providing a more accurate picture than any single Lewis structure could present. The real structure is a blend of these representations, often giving rise to a more stable arrangement than the contributing forms. This phenomenon highlights that electrons in molecules do not occupy fixed positions, but rather spread out, enhancing stability.
Resonance explains why certain bonds are stronger and more stable than anticipated based on simple Lewis structures.
The importance of recognizing resonance lies in its ability to influence the physical and chemical properties of the compound. Molecules exhibiting resonance tend to have lower energies, which translates to greater stability. This concept is not merely academic; rather, it impacts everything from molecular reactions to the pharmacological properties of drug candidates.
Resonance Forms and Stability
Resonance forms vary in contribution to the resonance hybrid. Some structures effectively represent the behavior of electrons more closely than others. Factors that affect the stability of resonance forms include:
- Number of bonds: More bonds typically equate to greater stability.
- Charge distribution: Structures that disperse charge more evenly tend to be favored.
- Electronegativity: Structures placing negative charges on more electronegative atoms are more stable.
The cumulative effect of these resonance forms creates a resonance hybrid that often results in bonds with characteristics between single and double bonds, known as partial bonds. Consequently, the compound exhibits traits such as increased bond strength and bond length variation directly related to these resonance dynamics. This understanding is fundamental in predicting how molecules will interact during chemical reactions.
Engaging with resonance structures lays the foundation for deeper insights into more complex chemical behavior, highlighting the intricate balance of stability and reactivity that defines structural chemistry.
Isomerism
Isomerism plays a critical role in the understanding of chemical compounds and their properties. It refers to the phenomenon where two or more compounds have the same molecular formula but differ in the arrangement of atoms. This slight variation can lead to significant differences in chemical behavior and reactivity. Isomers are categorized into various types, each contributing to the complexity and richness of chemistry.
The study of isomerism is essential for several reasons:
- Reactivity Differences: Isomers can react differently under similar conditions. This can influence reaction pathways and products.
- Bioactivity: In pharmacology, different isomers of a drug can exhibit vastly different therapeutic effects, making isomerism crucial for drug design.
- Physical Properties: Isomers may show variations in boiling points, melting points, and solubility due to differences in their structural configuration.
Greater awareness of isomerism is particularly important in fields like organic chemistry, material science, and biochemistry. Understanding the nuances of how isomers behave aids in predicting the outcomes of chemical reactions and the development of new materials.
Types of Isomers
Isomers can be classified mainly into two broad categories: structural isomers and stereoisomers. Each category further contains different specific types that highlight the range of possibilities in molecular arrangements.
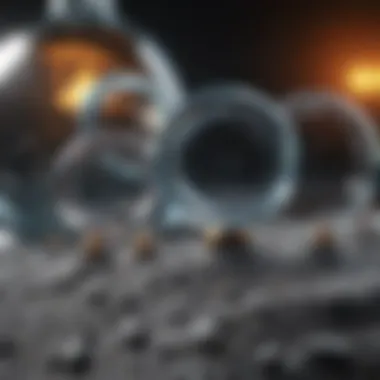
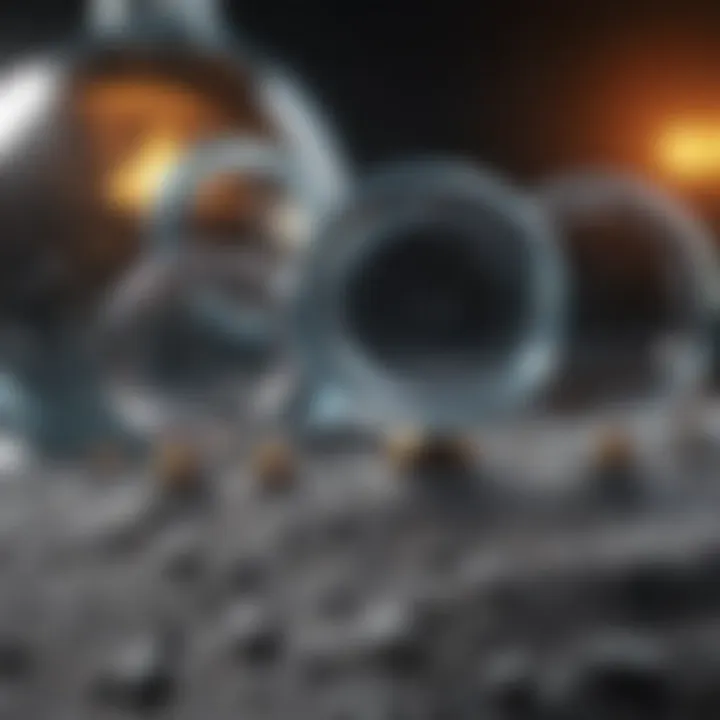
Structural Isomers
Structural isomers differ in the connectivity of atoms. Here are some examples:
- Chain Isomers: These isomers vary in the arrangement of the carbon skeleton. For instance, butane has two chain isomers: n-butane and isobutane.
- Position Isomers: These differ by the position of a functional group on the same carbon chain. An example includes butan-1-ol and butan-2-ol.
- Functional Group Isomers: These isomers have the same formula but different functional groups, such as ethanol and dimethyl ether.
Stereoisomers
Stereoisomers have the same connectivity but differ in the arrangement of their atoms in three-dimensional space. This category can be divided into:
- Geometric Isomers (Cis-Trans Isomers): These occur due to restricted rotation around double bonds or rings, leading to different spatial arrangements. For example, cis-2-butene and trans-2-butene.
- Optical Isomers (Enantiomers): These are non-superimposable mirror images of each other. A classic example is the two enantiomers of lactic acid, which can have very different biological activities.
Each type of isomer provides a unique perspective on how molecular structure influences chemical properties and behaviors.
Stereochemistry
Stereochemistry is the study of how the arrangement of atoms in space affects the behavior and properties of molecules. It plays an indispensable role in various fields including organic chemistry and medicinal chemistry. Understanding stereochemistry can explain why certain molecules behave differently despite having the same molecular formula.
Key aspects of stereochemistry include:
- Chirality: A molecule is chiral if it cannot be superimposed on its mirror image. Chirality often leads to enantiomers that can exhibit significantly different biological activities.
- Configuration: This refers to the spatial arrangement of atoms in a molecule. The configuration can be specified as R (rectus) or S (sinister) based on the Cahn-Ingold-Prelog priority rules.
- Conformational Isomers: These are different arrangements of atoms that are formed by rotation around single bonds. An example is the staggered and eclipsed conformations of ethane.
Understanding stereochemistry is valuable for chemists, particularly in drug design and development, as it provides insight into the interactions between molecules. Given that biological receptors are often chiral, the stereochemistry of a drug can greatly influence its efficacy.
"A deeper grasp of isomerism and stereochemistry is not just a theoretical exercise, but a foundation for innovations in chemistry and pharmacology."
Crystal Lattice Structures
The study of crystal lattice structures is pivotal in understanding the properties and behaviors of various compounds. A crystal lattice describes the orderly, repetitive arrangement of atoms, ions, or molecules in a crystalline material. This organization plays a significant role in determining many physical properties, including melting points, density, and even electrical conductivity. Knowledge of crystal structures allows chemists to predict how substances will interact in different conditions, which is crucial for both theoretical investigations and practical applications, such as materials science and drug design.
Key elements of crystal lattice structures include:
- Symmetry: The arrangement's symmetry can influence the physical properties.
- Unit Cells: The smallest repeating unit in the lattice, crucial for understanding the overall structure.
- Coordination Number: This indicates the number of nearest neighbors surrounding an atom, affecting interactions and stability.
Understanding these elements assists researchers in manipulating materials for desired outcomes.
Types of Crystal Lattices
Crystal lattices can be systematically categorized into a few distinct types, each with unique geometries and properties. The primary types of crystal lattices include:
- Simple Cubic Lattice: Atoms are positioned at the corners of a cube, offering a straightforward and less efficient packing structure.
- Body-Centered Cubic Lattice: Here, an additional atom is located at the center of the cube, enhancing packing efficiency compared to the simple cubic structure.
- Face-Centered Cubic Lattice: Atoms are found at each corner of the cube and at the center of each face, providing the highest packing efficiency among the simple lattices.
- Hexagonal Close-Packed Lattice: Atoms are arranged in a hexagonal formation, optimizing space and stability.
Each type of lattice exhibits distinct characteristics that influence the material's overall behavior. Understanding these types is essential for applications in various fields like metallurgy and crystallography.
Importance of Crystal Structures
Crystal structures are important for several reasons. They contribute to our understanding of material behaviors, allowing chemists and scientists to design new materials with specific properties. For example, in semiconductors, the crystal lattice structure is fundamental for electronic properties.
Additionally, crystal structures help in:
- Predicting Reactions: The arrangement impacts how substances will react with one another.
- Understanding Properties: Many macroscopic properties, such as strength and thermal conductivity, stem from the underlying crystal structure.
- Material Design: Tailoring the lattice structure can lead to innovations in drug formulations, catalysts, and other essential applications.
The study of crystal structures merges theoretical knowledge with practical applications, making it an indispensable aspect of structural chemistry.
In summary, crystal lattice structures provide a foundational framework for comprehending the complex behaviors of chemical compounds. Their significance extends beyond mere academic interest, impacting numerous scientific and industrial fields.
Spectroscopic Techniques
Spectroscopic techniques are essential tools in structural chemistry. They allow scientists to study the arrangement of atoms in chemical compounds and gain insights into their properties. Understanding these techniques helps in the elucidation of molecular structures, which is crucial for both theoretical knowledge and practical applications.
Spectroscopy relies on the interaction between matter and electromagnetic radiation. By observing these interactions, researchers can infer information about the chemical composition and molecular arrangement within a sample. Two of the most widely used spectroscopic methods are Nuclear Magnetic Resonance (NMR) spectroscopy and Infrared (IR) spectroscopy. Both techniques provide invaluable data when constructing the structural profiles of compounds.
NMR Spectroscopy
Nuclear Magnetic Resonance spectroscopy is a powerful analytical method used to determine the structure of organic compounds. This technique exploits the magnetic properties of certain atomic nuclei. When these nuclei are placed in a magnetic field, they resonate at a specific frequency. By measuring this resonance, scientists can gather data about the number of hydrogen atoms and their environment in a molecule.
In practice, NMR is often used to:
- Identify and quantify small organic molecules.
- Analyze complex mixtures.
- Determine the relative positions of atoms in a molecule, which helps with elucidating its structure.
Its non-destructive nature is a significant advantage. Researchers can obtain crucial information without altering the sample permanently. However, proper interpretation of NMR spectra demands a solid understanding of chemical shifts, spin-spin coupling, and integration values. These factors all play key roles in reinforcing the structural narrative that NMR provides.
IR Spectroscopy
Infrared spectroscopy is another fundamental technique widely used in structural chemistry. This method is based on the absorption of infrared radiation by molecular vibrational modes. When a molecule absorbs infrared light, it undergoes changes in its vibrational states, leading to unique spectral fingerprints that can be associated with functional groups within the compound.
IR spectroscopy proves beneficial for:
- Identifying functional groups in compounds.
- Analyzing molecular interactions.
- Studying changes in molecular structure under varying conditions.
One key point is that different bonds vibrate at characteristic frequencies, allowing for precise identification of organic molecules. However, distinguishing between similar functional groups can be complex and may require complementary methods, such as NMR or mass spectrometry, for complete analysis.
"Spectroscopic techniques illuminate the hidden details of molecular structures, shedding light on their chemical behavior and interactions."
Chemical Properties Related to Structure
Understanding the relationship between the structural composition of chemical compounds and their properties is crucial in the domain of chemistry. The structural frameworks dictate how molecules interact with one another and influence their physical and chemical behavior. Recognizing these patterns aids not only in theoretical studies but also in practical applications across various fields, including drug development, materials science, and environmental chemistry.
Polarity of Molecules
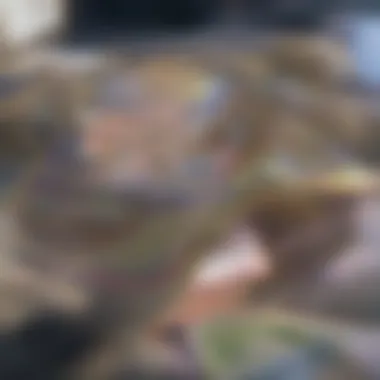
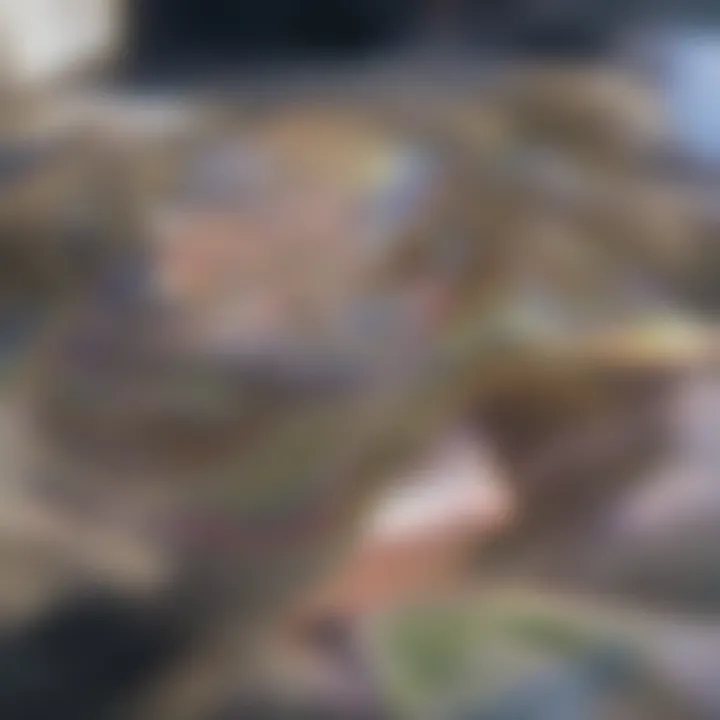
Polarity is a fundamental concept in chemistry that arises from the asymmetric distribution of electric charge within a molecule. This property primarily stems from the difference in electronegativity between the atoms involved in a bond. When atoms with different electronegativities form a bond, the electrons may not be shared equally. This can lead to a partial positive charge on one end of the molecule and a partial negative charge on the other end. The result is a dipole moment that can greatly affect the behavior of a substance.
- Importance of Polarity: Polarity plays an essential role in defining solubility, boiling points, and intermolecular interactions. Polar molecules tend to dissolve in polar solvents, while nonpolar molecules are typically soluble in nonpolar solvents. This principle is often summarized as "like dissolves like."
- Examples: Water is a classic example of a polar molecule. The oxygen atom attracts electrons more strongly than hydrogen atoms, causing a partial negative charge on the oxygen and a partial positive charge on the hydrogens. This polarity allows water to engage in hydrogen bonding, contributing to its high boiling point relative to its molecular weight.
"The polarity of a molecule not only influences its solubility but also its reactivity and interaction with other compounds."
Acidity and Basicity
Acidity and basicity, defined by the presence of protons (H+) and hydroxide ions (OH-) respectively, are inherently related to the structure of molecules. The structural features of a compound determine its ability to donate or accept protons, thus characterizing its acidic or basic nature.
- Structural Considerations: A molecule's functional groups significantly affect its acidity and basicity. For instance, carboxylic acids have a -COOH group that can easily release a proton, making them relatively strong acids. In contrast, alcohols, which contain a -OH group, are generally weaker acids due to the stability of their conjugate base.
- pKa Values: The strength of an acid is often conveyed through its pKa value, a numerical descriptor of acidity. Lower pKa values indicate stronger acids and are often aligned with structural features that stabilize the resulting conjugate base after a proton is lost.
The Role of Structural Chemistry in Drug Design
The role of structural chemistry in drug design is crucial. This field blends chemistry and biology to create effective pharmaceuticals. Understanding how molecular structures interact can lead to better drug design strategies. The molecular arrangement directly influences the efficacy, safety, and specificity of a drug. Consequently, researchers prioritize structural chemistry to optimize these factors, leading to the development of better therapeutic agents.
Importance of Molecular Interaction
Molecular interaction plays a key role in the drug discovery process. This interaction can occur between drug molecules and their biological targets, such as proteins or enzymes. A precise fit between a drug and its target results in better therapeutic outcomes. For instance, hydrogen bonds, ionic interactions, and van der Waals forces contribute to the binding process.
- Target Specificity: The design of molecules that can selectively bind to specific cellular targets reduces side effects. This leads to higher efficiency in treating diseases.
- Stability of Complexes: The strength of interactions helps maintain stable complexes. Stronger complexes typically result in prolonged action of medications.
- Predicting Behavior: Structural insights enable researchers to predict how drugs will behave in the body, enhancing the understanding of pharmacokinetics and pharmacodynamics.
"The success of drug design often hinges on the precise understanding of molecular interactions and their implications in biological systems."
Structure-Activity Relationship (SAR)
Structure-Activity Relationship (SAR) is a pivotal concept in medicinal chemistry. SAR examines how variations in molecular structure affect biological activity. By analyzing these relationships, researchers can identify functional groups that significantly impact the drug's potency. This method helps in optimizing lead compounds during the drug development process.
Key aspects of SAR include:
- Identifying Critical Groups: By altering certain chemical groups, researchers can observe resultant changes in activity. This aids in understanding which parts of a molecule are essential.
- Optimizing Lead Compounds: The information gained from SAR enables chemists to refine candidates and enhance their properties.
- Facilitating Synthesis: Understanding SAR can lead to more efficient synthetic routes when designing new compounds.
Successful application of SAR has paved the way for numerous advancements in pharmaceuticals. It is essential in aiming for faster and more effective drug development processes.
Challenges in Understanding Chemical Structures
Understanding chemical structures is essential for comprehending the principles that govern chemical behavior and interaction. However, the journey through the landscape of chemical compounds is fraught with challenges. This section highlights some key challenges researchers face while exploring chemical structures, particularly in organic chemistry and molecular modeling.
Complexity of Organic Compounds
Organic compounds often present significant challenges due to their vast diversity and complex behaviors. The sheer number of possible structures, influenced by arrangements of atoms, functional groups, and stereochemistry, creates an intricate tapestry for chemists to navigate.
- Diversity: Organic compounds can vary significantly in structure, which leads to different properties and reactivities. The presence of different functional groups can contribute to a compound's characteristics and behavior.
- Stereochemistry: Understanding the three-dimensional arrangement of atoms is crucial. Enantiomers, for example, can have vastly different physiological effects, complicating the evaluation of a compound's behavior.
- Predictive Models: Many molecules defy simple models and require advanced techniques to predict behaviors based on structure. This can require sophisticated analysis and experimentation, leading to difficulties in deriving universal rules.
Research surrounding organic compounds emphasizes the critical importance of understanding their complexities. Only through appreciation of these intricacies can researchers innovate and apply their knowledge effectively.
Limitations in Molecular Modeling
Molecular modeling serves as a pivotal tool in the analysis of chemical structures. However, several limitations can hinder its effectiveness. The challenges associated with molecular modeling often stem from computational resources and the simplifications involved in simulations.
- Computational Limitations: High-level quantum calculations can be resource-intensive. This poses limitations on the size and complexity of molecules that can be accurately simulated. Often, approximations must be made, which can lead to less precise predictions.
- Simplified Representations: Molecular models often rely on simplified representations. These models may not fully capture the nuances of molecular interactions, especially in dynamic environments where temperature and pressure influence behavior.
- Parameterization Issues: Many models depend on empirical parameters derived from experimental data. If data is limited or flawed, the models derived from them may also be inaccurate, leading to misunderstandings of actual molecular behaviors.
Ultimately, understanding these limitations helps researchers approach studies with a more critical eye. Progress in computational chemistry continues to address these challenges, refining the techniques available and broadening the scope of potential discoveries in the field.
"The structures of organic compounds reveal much about their identity and reactivity, yet unraveling their complexities remains a significant challenge."
Future Directions in Structural Chemistry
The field of structural chemistry is evolving rapidly. As we push the boundaries of our understanding, new methodologies enhance our capacity to analyze and characterize chemical compounds. Future directions focus on integrating technology, refining theoretical models, and expanding research to cover complex molecular systems. This ongoing evolution is critical because it directly affects various applications across industries, from pharmaceuticals to materials science.
Advancements in Computational Chemistry
Computational chemistry represents a significant advancement in structural chemistry. It provides tools for simulating molecular behavior, enabling scientists to predict properties and reactivities before physical experiments. Quantum mechanical calculations help elucidate the electronic structure of molecules, offering insights into their stability and potential reactions.
Key benefits include:
- Efficiency: Computers can process complex calculations much faster than traditional methods.
- Safety: Simulating reactions virtually reduces the need for potentially hazardous laboratory experiments.
- Cost-effectiveness: Computational models can minimize the resources needed for physical trials, leading to significant savings.
Tools such as density functional theory (DFT) and molecular dynamics simulations allow for the exploration of chemical spaces that were previously inaccessible. As computer hardware improves and algorithms become more sophisticated, the accuracy and applicability of computational chemistry will only increase.
Integrating Structural Analysis with Big Data
The incorporation of big data analytics in structural chemistry is a relatively new frontier but has vast potential. This approach involves analyzing extensive datasets derived from various sources, including experimental results, theoretical predictions, and even real-time data from spectroscopic methods. By employing machine learning algorithms, chemists can identify patterns and correlations that traditional methods might overlook.
Benefits of this integration include:
- Enhanced predictive power: Big data tools can improve the prediction of molecular properties and behaviors by considering a broader range of variables.
- Faster discovery: Automating data analysis allows for quicker insights in research and development phases.
- Better collaboration: Sharing large datasets across research institutions enhances collective knowledge and accelerates advancements in the field.
Finale
- Understanding molecular geometry is essential. It allows chemists to predict how molecules will interact with each other, which is vital in fields like drug design and materials science.
- Chemical bonding determines the strength and nature of interactions between atoms. Recognizing the differences between ionic, covalent, and metallic bonds helps explain various physical and chemical properties.
- Functional groups provide insight into the reactivity of organic compounds. This knowledge enables chemists to devise new materials and find innovative applications.
- Isomerism and stereochemistry are crucial for understanding how slight variations in molecular structure can lead to significant differences in behavior and reactivity. This aspect is especially important in pharmaceuticals, where specific isomers can have entirely different effects on biological systems.
- Resonance structures further illustrate the dynamic nature of chemical compounds, allowing for a more accurate depiction of electron distribution.
The intricate interplay among these elements forms a complex web that defines chemical behavior. Recognizing these connections is critical for advancing both theoretical and applied chemistry.
Summary of Key Points
Throughout the article, we have highlighted several fundamental aspects:
- The significance of molecular arrangement and its impact on chemical behavior.
- Different types of chemical bonding and their implications for stability and reactivity.
- The role of functional groups in dictating the chemical properties of organic compounds.
- The complexities of isomerism and its influence on stereochemistry.
- The relevance of resonance structures in understanding electronic configurations of molecules.
Implications for Future Research
The structural chemistry field is evolving, opening up new avenues for research and discovery. Future inquiries may focus on:
- Computational advancements: As technology improves, so does our ability to model complex molecular structures more accurately. This can lead to better predictions of chemical properties and behaviors.
- Integration with big data: The merger of structural analysis with big data approaches can provide unprecedented insights into chemical relationships, enhancing material design and drug development.
- Sustainable chemistry: Examining how structure influences environmental impact could drive the development of greener chemicals and processes.
Understanding the structural foundations will continue to be critical as we venture into next-generation materials and innovative therapies. The knowledge gained from studying chemical structures not only enriches the scientific community but also holds practical implications that can transform various industries.